Crosstalk between cancer cell plasticity and immune microenvironment in cholangiocarcinoma
Abstract
Cholangiocarcinoma (CCA) is a highly aggressive tumor of the biliary tree characterized by an intense desmoplastic tumor microenvironment (TME). To date, treatment of CCA remains challenging; tumor resection is the only curative treatment with a high recurrence probability. Besides resection, therapeutic options have moved forward with the advent of immunotherapies, but these remain limited and low effective. Our knowledge about the cellular interplays in CCA is still fragmentary. An area is currently emerging regarding the potential role of cancer cell plasticity in the genesis of an immunosuppressive microenvironment. The cancer cells’ ability to acquire stemness properties and to disseminate through an epithelial-mesenchymal transition (EMT) shape a tumor immune microenvironment that supports cancer progression by attracting immunosuppressive cells including myeloid-derived suppressor cells (MDSCs), regulatory T cells (Tregs), M2 macrophages, and by increasing the expression of inhibitory immune checkpoints such as PD-1/PD-L-1. EMT-inducing transcription factors (EMT-TF) have recently emerged as regulators of tumor immunity by creating an immunosuppressive microenvironment. This review delves into the molecular mechanisms underlying the existing links between EMT/stemness and tumor immune microenvironment, as well as the last discoveries in CCA.
Keywords
INTRODUCTION
The great adaptability of cancer cells in nature confers cellular plasticity and heterogeneity inside the tumor[1]. Cell plasticity in cancer is an adaptive mechanism irrespective of genetic alterations by which cancer cells modify their phenotype in response to external cues. Owing to cellular plasticity, cancer cells acquire pro-invasive capabilities and stem cell properties, stimulate an immunosuppressive microenvironment, and resist anti-cancer treatments. The best-known plasticity process is the EMT, which prompts cells to transition from an epithelial phenotype to a mesenchymal one, contributing to tumor progression and dissemination[2]. Cells undergoing EMT can also acquire stemness traits favoring tumor persistence and drug resistance. Indeed, EMT is also considered a defense mechanism that endows cancer cells with an advantage in proliferation, motility, and resistance to drugs, including immunotherapies. Beyond current knowledge on cell plasticity, recent studies strongly indicate that this ability enables cancer cells to escape the immune system through mechanisms that start to be elucidated. Through cell plasticity, tumor cells contribute to the establishment of an immunosuppressive TME, limiting the immune control of the tumor[3]. The interconnection between cell plasticity and immune evasion involves signals from cancer cells, and from both cellular and acellular components of TME. Among these signals, intracellular and extracellular factors already identified as major regulators of EMT and stemness contribute to abolishing the anti-immune response. Soluble factors such as the prototypal cytokine-induced EMT, transforming growth factor-β1 (TGF-β1), EMT-inducing transcription factors (EMT-TFs) including zinc finger E-box binding homeobox 1/2 (ZEB1/2), Snail family transcriptional repressor 1/2 (SNAI1/2) and TWIST family bHLH transcription factor 1/2 (TWIST 1/2), and epigenetic factors have been involved in the regulation of immune response in several carcinomas by remodeling the TME. The purpose of this review is to provide an overview of two major pathophysiological processes that are obviously intertwined in cancer, i.e., cell plasticity and immunobiology.
EPITHELIAL-MESENCHYMAL TRANSITION AND TUMOR IMMUNE MICROENVIRONMENT
EMT is a multi-step cellular plasticity program essential for embryonic development and organogenesis, which is also critical for cancer initiation, progression, and metastasis[4]. During EMT, epithelial cancer cells progressively lose their apico-basal polarity and cell junctions to acquire mesenchymal cell traits characterized by the expression of extracellular matrix (ECM) remodeling components and higher motility. All these events endow the cancer cells with spread properties into the adjacent tissue and distance into secondary tissues. As a dynamic reversible process, EMT generates a continuum of cells with intermediate, hybrid epithelial, and mesenchymal phenotypes. Cancer cell plasticity through EMT shapes a tumor immune microenvironment favorable to cancer progression by attracting immunosuppressive cells including myeloid-derived suppressor cells (MDSC), Tregs, M2 macrophages, and by increasing the expression of inhibitory immune checkpoints such as PD-1/PD-L-1. Correlative immunohistochemical analyses of EMT and immune markers in a large diversity of human solid cancers are in favor of an interplay between cell plasticity and immunosuppressive TME. In lung adenocarcinomas that display an EMT phenotype, the mesenchymal status of the tumor cells correlates with an increased expression of immune checkpoint molecules, including PD-L1, PD-L2, PD-1, TIM-3, B7-H3 (CD276), BTLA and CTLA-4[5]. Similarly, the expression of the metalloproteinase MMP-13 and TWIST1, two EMT markers, is associated with the expression of CTLA-4/PD-1/PD-L1/TIM-3/LAG-3 in esophageal squamous cell carcinoma[6]. In ovarian, prostate, and lung cancers, EMT signature correlates with a higher expression of immune checkpoint proteins, increased infiltration of immunosuppressive cells Tregs and M2 macrophages, and a lower CD8+ T cells infiltration[5,7-9]. A pan-cancer EMT analysis of The Cancer Genome Atlas (TCGA) dataset has evidenced that EMT-high tumors (mesenchymal-like) have a higher immunosuppressive TME compared to EMT-low (epithelial-like) tumors[7]. EMT-high tumors are distinguished by the infiltration of tumor-associated macrophages (TAM), the overexpression of immune checkpoint molecules, the upregulation of immune inhibitory cytokines TGF-β1 and IL10, and the enrichment of inflammatory and exhausted CD8+ T-cell signatures[10,11]. Similar results were found in colorectal cancer (CRC). In conclusion, according to several cancer analyses from public and non-public datasets, there is a positive interdependence between the EMT status of a tumor and its immunosuppressive nature. This correlative statement may serve to identify potential biomarkers and to stratify patients for personalized treatments with immune checkpoint inhibitors or other immunotherapy approaches.
Mechanistically, EMT is governed by a panel of transcription factors (i.e., EMT-TF) that regulate the expression of epithelial and mesenchymal markers, in negative and positive ways, respectively. The most known EMT-TFs include SNAl1/2, ZEB1/2, and TWIST1/2. Besides transcription factors, tumor cells produce many different soluble autocrine and paracrine factors that trigger EMT directly or indirectly via the stromal (CAF) or immune cells[12]. For instance, TGF-β1 is a pleiotropic cytokine with multiple biological effects in cancer, either an antitumoral role in the early stages of carcinogenesis, or a pro-tumoral function in later stages. In the latter case, the cytokine is by far a well-known EMT inducer produced by both tumor and TME cells[12]. TGF-β1 signaling drives tumor cell plasticity by inducing mesenchymal features along with stemness traits, leading to cell invasion and resistance to anti-cancer drugs. In addition, TGF-β1 shapes an immune-suppressive TME by upregulating the expression of immune checkpoints and attracting immunosuppressive cells. TGF-β1 may also affect immune surveillance by decreasing immunoproteasome, which is a process generating peptides binding to HLA I molecules to facilitate antigen presentation to CD8+ T cells[13]. Extracellular cues emerging from acellular components of TME also regulate EMT. Indeed, ECM molecules or ECM remodeling proteins activate intracellular signaling pathways followed by EMT-TF activation to drive EMT[12]. The underlying molecular mechanisms at the origin of the interplay between EMT and immunosuppression have partly been identified. SNAI1, ZEB1, and TWIST1 are transcription factors governing EMT that also regulate antitumor immune responses. Beyond the regulation of the expression of epithelial and mesenchymal markers, these transcription factors regulate the expression of inhibitory immune checkpoints and soluble factors that attract immunosuppressive cells[14]. A summary of these regulations is provided in the next chapter.
REGULATION OF TUMOR IMMUNE MICROENVIRONMENT BY EPITHELIAL-MESENCHYMAL TRANSITION-INDUCING TRANSCRIPTION FACTORS
SNAI1
SNAI1 regulates the expression of thrombospondin 1 (TSP1) in melanoma cells, which is a secreted matrix protein that promotes Tregs infiltration and impairs dendritic cell (DC) functions[15]. Cancer cells expressing SNAI1 release chemokine ligand 2 (CCL2)/monocyte chemoattractant protein 1, (MCP1) and lipocalin 2 (LCN2). Together, CCL2 and lipocalin 2 stimulate regulatory dendritic cells (DCreg), which display an immunosuppressive activity, by decreasing the expression of costimulatory molecules (e.g., HLA-DR) and increasing the expression of immunosuppressive molecules (e.g., PD-L1) in human peripheral blood mononuclear cells (PBMCs). The CCL2/LCN2-induced DCreg cells subsequently trigger the proliferation of immunosuppressive CD4+/FOXP3+ Treg cells and impair tumor-specific cytosolic T cells (CTL) induction[16]. In breast cancer cells, SNAI1 induces mesenchymal and stemness features that alter the susceptibility of cancer cells to T-cell–mediated immune surveillance through an autophagy mechanism[17]. Additionally, SNAI1 stimulates the expression of PD-L1 at the surface of breast cancer cells via a post-translational upregulation of proteins containing the CKLF-like MARVEL transmembrane (CMTM) 6 and 7, leading to immune evasion[18]. In the transgenic MMTV-PyMT mouse model of breast adenocarcinoma, SNAI1high mesenchymal cancer cells exhibited an increased Tregs infiltration and a M2 macrophage polarization compared to SNAI1low epithelial cancer cells and displayed high resistance to anti-CTLA-4 treatment[19]. Overexpression of SNAI1 or ZEB1 in breast cancer cells leads to an increased expression of CD47, a macrophage immune checkpoint protein also considered as a “don’t eat me” signal that suppresses macrophage phagocytic activity, preventing cancer cell death from macrophages. It has been shown that both transcription factors SNAI1 and ZEB1 directly bind to two E-boxes located on the CD47 gene promoter. The analysis of ChIP-seq data performed in mice breast tumors showed that SNAI1 can bind to many more genes; 89 immunomodulatory genes were identified, including the promoters of Nt5e (coding CD73), Csf1 and Spp1 genes[20]. SNAI1 regulates the expression of other immunosuppressive factors including CXCL1 and CXCL2, two chemokines that attract the immunosuppressive cells MDSCs to the tumor via CXCR2[21]. Post-translational modification of SNAI1 by CREB-binding protein (CBP) prevents the repressor complex formation and induces the transcription of TNFA, CCL2, and CCL5, promoting the recruitment of TAM and tumor progression[22]. In humans, analysis of TCGA and METABRIC data sets from breast cancer patients highlighted a correlation between CD47, SNAI1, and vimentin[23].
ZEB1
In breast cancer and non-small-cell lung cancer (NSCLC) cells, ZEB1 upregulates PD-L1 expression by downregulating miR-200, a well-known repressor of PD-L1 expression[11]. By indirectly upregulating PD-L1, ZEB1 leads to the exhaustion of CD8+ T cells[11]. However, ZEB1 has been shown to directly regulate PD-L1 expression by binding its gene promoter that contains a ZEB1 binding E-box[24]. In colorectal cancer, expression of ZEB1 positively correlates with PD-L1[25]. In patients, co-expression of ZEB1 and PD-L1 is associated with a higher cervical lymph node metastasis and a lower survival rate in oral squamous cell carcinoma[26]. ZEB1 also induces the expression of CD47 in cancer-invading cells (i.e., mesenchymal-like cells), driving the M2 polarization of adjacent TAMs in the lung adenocarcinoma mouse model. Thus, ZEB1 reprograms the immune microenvironment surrounding invading clusters[24]. ZEB1 impacts CD70 expression in lung cancer mesenchymal cell lines; CD70 belongs to the tumor necrosis factor (TNF) superfamily, and its expression in T cells limits their expansion. In mesenchymal NSCLC patient samples, CD70 is overexpressed and positively associated with decreased CD3+ and CD8+ T-cell infiltration and increased T-cell exhaustion markers[27]. Recently, Caramel and Coll. have nicely demonstrated that ZEB1 expression in melanoma cells is associated with a decreased CD8+ T-cell infiltration. They show that ZEB1 directly represses the secretion of T cell-attracting chemokines, including CXCL10, leading to resistance against immune checkpoint inhibitors[28].
TWIST1
TWIST1 has been shown to regulate CSF1 (Colony-stimulating factor-1/MCSF), a TAM chemotactic molecule, through a transcriptional regulation since CSF1 promotor contains TWIST responsive elements[29]. Biologically, TWIST1 increases the expression of CSF1, inducing an activation and polarization of TAM[29]. In addition to CSF1, TWIST1 regulates CCL2 in human mammary epithelial cells, promoting the attraction of macrophages in vitro and their infiltration in vivo[30]. In a murine model of MYC-driven hepatocellular carcinoma (HCC), TWIST1 cooperates with MYC to induce a transcriptional program that stimulates the expression of CCL2 and IL13, leading to the recruitment and polarization of TAM[31].
In conclusion, several EMT-TFs directly or indirectly initiate transcriptional programs that promote the expression of molecules at the origin of an immune permissive TME.
STEMNESS AND TUMOR IMMUNE MICROENVIRONMENT
Cancer stem cells (CSCs) are a key component of TME that play important roles in cancer initiation and progression, and therapy resistance. A strong link between CSC and the immune ecosystem has recently been highlighted[32,33]. Several immunohistochemistry studies on human samples have demonstrated a good correlation between stemness and immunity markers in tumors. Based on a signature of five stemness-related genes, a stemness-related prognostic index (SPI) was calculated in the head and neck squamous cell carcinomas (HNSCC). It was shown that the high-SPI group with poor prognosis had a “cold” immunologic profile, characterized by an increased number of TAMs and CAFs, and a low level of CD8+ T and B cells. On the opposite, the SPI-low group of HNSCC displays an increased number of CD8+ T cells, aligning the characteristic of inflamed/mesenchymal subtype[34]. Based on comprehensive bioinformatic analyses, it has been shown that CRC patients with higher stemness scores exhibited a worse prognosis, a higher immunosuppressive TME, and lower immunotherapeutic responses[35]. Several explanations may justify the link between cancer stem cells and immunosuppressive TME. It is known that CSCs express a higher level of PD-L1 than cancer cells, with the existence of a positive association between CSC expansion and a high PD-L1 expression in TME. CSCs also express B7.1 (CD80), another antitumor checkpoint, and CD47. Together, PD-L1, CD80, and CD47 prevent an antitumor immune response. CSCs are also characterized by a low expression of major histocompatibility complex-1 (MHC-1) molecules or antigen processing and presentation machinery, which contributes to lower immunogenicity in these cells, enabling CSCs to escape the immune system[36,37]. In summary, CSCs regulate the TME by inhibiting the antitumor immunity; they suppress CD8+ T cell infiltration and promote the recruitment of type 2 macrophages (M2). Furthermore, by recruiting macrophages, CSCs build a niche for maintaining their stemness features[38].
EMT, STEMNESS, AND IMMUNE MICROENVIRONMENT IN CHOLANGIOCARCINOMA
CCA contains a high contingency of CSCs, i.e., 30%, and many cancer cells express epithelial and mesenchymal markers[39-42]. Although a link between EMT and stemness is well established in this tumor with major consequences on tumor progression and drug resistance[43-45], there are few studies related to cancer cell plasticity and immune evasion in CCA. The first study is related to atypical protein kinase C-iota (aPKC-ι)[46]. Overexpression of aPKC-ι in malignant CCA cells promotes EMT by upregulating SNAI1 expression via the phosphorylation of the transcription factor Sp1, providing cells with mesenchymal-like features. Within a mesenchymal phenotype, tumor cells indirectly induce Treg‐like cells through other immune cells or by secreting immunosuppressive cytokines, partly mediated by Treg‐inducible cytokines such as IL‐2 and TGF‐β1[46]. More recently, it has been demonstrated that CCA malignant cells with high stemness traits express a lower level of MHC-2 molecules than tumor cells with low stemness features[37]. In addition, high stemness malignant cells express high levels of inflammatory factors, contributing to immune evasion[47].
Mutual crosstalk between CSCs and TAMs has been emphasized in CCA[48-51]. The two cell types are important CCA cell contingents comprising 30% CSCs and 10% TAMs[39,52]. Recently, it has been demonstrated that CSCs derived from CCA cell lines (HuCC-T1 or SB1) express CD11b, a surface marker for macrophages, leading to increased infiltration of macrophages in CSCs-derived tumors in immunodeficient mice[49]. CSCs from CCA also produce soluble molecules involved in the differentiation, activation, and recruitment of macrophages[48], enabling the formation of a tumorigenic niche essential for maintaining CSC characteristics[38,49]. Among these molecules, IL13, IL34 and osteoactivin have been identified in the CSC secretome at the origin of macrophagic-based niche[48]. Furthermore, macrophages produce and secrete TGF-β1 promoting EMT in CCA cells via a Smad2/3-GLI2 signaling pathway[50,53]. As previously mentioned, TGF-β1 is a prototypic inducer of EMT, which displays immunosuppressive properties[54]. In addition to macrophages, CAFS produce pleiotropic factors attracting immune suppressive cells such as MDSCs. By recruiting MDSCs through a 5-LO/LTB4-BLT2 axis, CAFs contribute to increasing the stemness of malignant cells[55,56].
With the advent of single-cell RNA sequencing, dissecting the TME of CCA has shed light on information regarding TME cell populations and malignant cell heterogeneity. A molecular classification of intrahepatic-CCA (iCCA) based on stroma, tumor, and immune microenvironment elements has evidenced a hepatic stem-like class enriched in M2-like macrophage[51]. In peripheral small duct type iCCA (iCCApps), tumor cells positive for the inhibitor of DNA binding and differentiation 3 (ID3) were identified; ID3 is known to contribute to the acquisition of a molecular stem cell-like signature[57]. Along with ID3, tumor cells express several stemness markers, such as ZEB1 or LGR5. Even if no correlative studies between ID3+ and immune cells were performed in this work, iCCApps containing CD3+ cells display an infiltration of CD3+ T cells and CD56+ NK cells, less M2-like phenotype macrophages and a better prognosis[57], suggesting that in this specific subtype of iCCA, stemness phenotype is linked to a better antitumor immunity. Of note, further studies are needed to confirm this speculative conclusion.
In another cancer of the biliary epithelium, gallbladder cancer, the inhibitory immune checkpoints CD73 and PD-L1 are closely associated with both EMT and stemness phenotypes. Knockdown of CD73 or PD-L1 reduced the proliferative and migratory functions of cancer cells, suggesting that CD73 and PD-L1 are two potential targets to consider as therapeutic strategies for treating tumors containing high populations of cells with CSC and EMT features[58].
CONCLUSION
Cell plasticity refers to the ability of cells to change their identity and behavior in response to different cues or stimuli. This phenomenon is particularly relevant in cancer, as it plays a crucial role in tumor progression, metastasis, and therapeutic resistance. Cancer cells can undergo various forms of plasticity, allowing them to adapt to different microenvironments and evade the body's immune system. In CCA, the immune landscape is the subject of numerous studies and reviews that describe the immune cells in partnership with other TME cells at the origin of the immunosuppressive environment[59-61]. EMT is a key process associated with malignant cell plasticity in tumors. EMT enables malignant epithelial cells to acquire mesenchymal properties, such as enhanced mobility and invasiveness. These transitions facilitate the invasion of cancer cells into surrounding tissues, dissemination to distant sites, and the formation of secondary tumors through a reverse process called mesenchymal to epithelial transition (MET). Besides tumor dissemination, EMT represents a non-genetic adaptive pathway for cells to survive upon therapeutic pressure and to escape the immune system, both adaptive and innate.
EMT can confer stemness properties to cancer cells, which may also contribute to the loss of immunosurveillance. Thus, cell plasticity including EMT or stemness has become a crucial biological way for malignant cells to evade the anti-cancer immune system. The molecular mechanisms behind this process have started to be elucidated, but this domain of research remains in its early stages in CCA. However, the strong relationship established between cell plasticity and immune TME may have an impact on clinical implications for prognosis evaluation. Indeed, the presence of cancer cells with mesenchymal or CSC markers could be a sign of an immunosuppressive TME by the work of Job et al., in which they classify iCCA according to a stromal signature[62]. The authors established a classification of four immune subtypes. Interestingly, the fourth subtype, which is not an immunogenic subtype, displays a signature of CSC, EMT, and TGF-β1 signaling[62]. This classification is therefore important for selecting proper treatments and considering combinatorial therapies. Currently, an immune checkpoint inhibitor, durvalumab, combined with chemotherapy, is administered to CCA patients. Other combination treatments could be considered, such as metronidazole, which reduces the number of stem cells in preclinical models of CCA[63]. Further information on various therapeutic approaches to eradicate cancer stem cells in liver is well discussed in this review[41]. Strategies targeting EMT regulators could form part of the therapeutic arsenal to reduce the mesenchymal characteristics of cancer cells undergoing EMT and enhance the efficacy of immune checkpoint inhibitors. For instance, anti-vimentin therapies seem promising[64]; in the case of CCA, this could be relevant since vimentin is highly expressed[39,65]. In conclusion, little is known about cellular plasticity in CCA and its link with the immune microenvironment. A better understanding of this link could lead to improved CCA patient management, in particular by proposing more effective combination therapies.
DECLARATIONS
Authors’ contributions
Wrote the first version of the article: Fouassier L
Edited and approved the final version: Minini M, Pavy A, Lekbaby B
Availability of data and materials
Not applicable.
Financial support and sponsorship
Minini M and Fouassier L received financial support from ITMO Cancer of Aviesan within the 2021-2030 Cancer Control Strategy framework on funds administered by Inserm.
Conflicts of interest
All authors declared that there are no conflicts of interest.
Ethical approval and consent to participate
Not applicable.
Consent for publication
Not applicable.
Copyright
© The Author(s) 2024.
REFERENCES
1. Saha S, Pradhan N, B N, Mahadevappa R, Minocha S, Kumar S. Cancer plasticity: investigating the causes for this agility. Semin Cancer Biol 2023;88:138-56.
2. Pérez-González A, Bévant K, Blanpain C. Cancer cell plasticity during tumor progression, metastasis and response to therapy. Nat Cancer 2023;4:1063-82.
3. Gu Y, Zhang Z, Ten Dijke P. Harnessing epithelial-mesenchymal plasticity to boost cancer immunotherapy. Cell Mol Immunol 2023;20:318-40.
4. Fares J, Fares MY, Khachfe HH, Salhab HA, Fares Y. Molecular principles of metastasis: a hallmark of cancer revisited. Signal Transduct Target Ther 2020;5:28.
5. Lou Y, Diao L, Cuentas ER, et al. Epithelial-mesenchymal transition is associated with a distinct tumor microenvironment including elevation of inflammatory signals and multiple immune checkpoints in lung adenocarcinoma. Clin Cancer Res 2016;22:3630-42.
6. Mahmoudian RA, Mozhgani S, Abbaszadegan MR, Mokhlessi L, Montazer M, Gholamin M. Correlation between the immune checkpoints and EMT genes proposes potential prognostic and therapeutic targets in ESCC. J Mol Histol 2021;52:597-609.
7. Mak MP, Tong P, Diao L, et al. A patient-derived, pan-cancer EMT signature identifies global molecular alterations and immune target enrichment following epithelial-to-mesenchymal transition. Clin Cancer Res 2016;22:609-20.
8. Kolijn K, Verhoef EI, Smid M, et al. Epithelial-mesenchymal transition in human prostate cancer demonstrates enhanced immune evasion marked by IDO1 expression. Cancer Res 2018;78:4671-9.
9. Hu Z, Cunnea P, Zhong Z, et al. The Oxford classic links epithelial-to-mesenchymal transition to immunosuppression in poor prognosis ovarian cancers. Clin Cancer Res 2021;27:1570-9.
10. Tiwari JK, Negi S, Kashyap M, Nizamuddin S, Singh A, Khattri A. Pan-cancer analysis shows enrichment of macrophages, overexpression of checkpoint molecules, inhibitory cytokines, and immune exhaustion signatures in emt-high tumors. Front Oncol 2021;11:793881.
11. Chen L, Gibbons DL, Goswami S, et al. Metastasis is regulated via microRNA-200/ZEB1 axis control of tumour cell PD-L1 expression and intratumoral immunosuppression. Nat Commun 2014;5:5241.
12. Zhang J, Hu Z, Horta CA, Yang J. Regulation of epithelial-mesenchymal transition by tumor microenvironmental signals and its implication in cancer therapeutics. Semin Cancer Biol 2023;88:46-66.
13. Tripathi SC, Peters HL, Taguchi A, et al. Immunoproteasome deficiency is a feature of non-small cell lung cancer with a mesenchymal phenotype and is associated with a poor outcome. Proc Natl Acad Sci U S A 2016;113:E1555-64.
14. Taki M, Abiko K, Ukita M, et al. Tumor immune microenvironment during epithelial-mesenchymal transition. Clin Cancer Res 2021;27:4669-79.
15. Kudo-Saito C, Shirako H, Takeuchi T, Kawakami Y. Cancer metastasis is accelerated through immunosuppression during Snail-induced EMT of cancer cells. Cancer Cell 2009;15:195-206.
16. Kudo-Saito C, Shirako H, Ohike M, Tsukamoto N, Kawakami Y. CCL2 is critical for immunosuppression to promote cancer metastasis. Clin Exp Metastasis 2013;30:393-405.
17. Akalay I, Janji B, Hasmim M, et al. Epithelial-to-mesenchymal transition and autophagy induction in breast carcinoma promote escape from T-cell-mediated lysis. Cancer Res 2013;73:2418-27.
18. Xiao M, Hasmim M, Lequeux A, et al. Epithelial to mesenchymal transition regulates surface PD-L1 via CMTM6 and CMTM7 induction in breast cancer. Cancers 2021;13:1165.
19. Dongre A, Rashidian M, Reinhardt F, et al. Epithelial-to-mesenchymal transition contributes to immunosuppression in breast carcinomas. Cancer Res 2017;77:3982-9.
20. Dongre A, Rashidian M, Eaton EN, et al. Direct and indirect regulators of epithelial-mesenchymal transition-mediated immunosuppression in breast carcinomas. Cancer Discov 2021;11:1286-305.
21. Taki M, Abiko K, Baba T, et al. Snail promotes ovarian cancer progression by recruiting myeloid-derived suppressor cells via CXCR2 ligand upregulation. Nat Commun 2018;9:1685.
22. Hsu DS, Wang HJ, Tai SK, et al. Acetylation of snail modulates the cytokinome of cancer cells to enhance the recruitment of macrophages. Cancer Cell 2014;26:534-48.
23. Noman MZ, Van Moer K, Marani V, et al. CD47 is a direct target of SNAI1 and ZEB1 and its blockade activates the phagocytosis of breast cancer cells undergoing EMT. Oncoimmunology 2018;7:e1345415.
24. Guo Y, Lu X, Chen Y, et al. Zeb1 induces immune checkpoints to form an immunosuppressive envelope around invading cancer cells. Sci Adv 2021;7:eabd7455.
25. Xu J, Yang X, Pan J, Fan H, Mei J, Hua D. Biochanin A suppresses tumor progression and PD-L1 expression via inhibiting ZEB1 expression in colorectal cancer. J Oncol 2022;2022:3224373.
26. Takamaru N, Fukuda N, Akita K, Kudoh K, Miyamoto Y. Association of PD-L1 and ZEB-1 expression patterns with clinicopathological characteristics and prognosis in oral squamous cell carcinoma. Oncol Lett 2022;23:75.
27. Ortiz-Cuaran S, Swalduz A, Foy JP, et al. Epithelial-to-mesenchymal transition promotes immune escape by inducing CD70 in non-small cell lung cancer. Eur J Cancer 2022;169:106-22.
28. Plaschka M, Benboubker V, Grimont M, et al. ZEB1 transcription factor promotes immune escape in melanoma. J Immunother Cancer 2022;10:e003484.
29. da Silva SD, Marchi FA, Su J, et al. Co-overexpression of TWIST1-CSF1 is a common event in metastatic oral cancer and drives biologically aggressive phenotype. Cancers 2021;13:153.
30. Low-Marchelli JM, Ardi VC, Vizcarra EA, van Rooijen N, Quigley JP, Yang J. TWIST1 induces CCL2 and recruits macrophages to promote angiogenesis. Cancer Res 2013;73:662-71.
31. Dhanasekaran R, Baylot V, Kim M, et al. MYC and TWIST1 cooperate to drive metastasis by eliciting crosstalk between cancer and innate immunity. Elife 2020;9:e50731.
32. Rouzbahani E, Majidpoor J, Najafi S, Mortezaee K. Cancer stem cells in immunoregulation and bypassing anti-checkpoint therapy. Biomed Pharmacother 2022;156:113906.
33. Sipos F, Műzes G. Cancer stem cell relationship with pro-tumoral inflammatory microenvironment. Biomedicines 2023;11:189.
34. Luo Y, Xu WB, Ma B, Wang Y. Novel stemness-related gene signature predicting prognosis and indicating a different immune microenvironment in HNSCC. Front Genet 2022;13:822115.
35. Zheng H, Liu H, Li H, et al. Characterization of stem cell landscape and identification of stemness-relevant prognostic gene signature to aid immunotherapy in colorectal cancer. Stem Cell Res Ther 2022;13:244.
36. Yang W, Li Y, Gao R, Xiu Z, Sun T. MHC class I dysfunction of glioma stem cells escapes from CTL-mediated immune response via activation of Wnt/β-catenin signaling pathway. Oncogene 2020;39:1098-111.
37. Di Tomaso T, Mazzoleni S, Wang E, et al. Immunobiological characterization of cancer stem cells isolated from glioblastoma patients. Clin Cancer Res 2010;16:800-13.
38. Luo S, Yang G, Ye P, et al. Macrophages are a double-edged sword: molecular crosstalk between tumor-associated macrophages and cancer stem cells. Biomolecules 2022;12:850.
39. Cardinale V, Renzi A, Carpino G, et al. Profiles of cancer stem cell subpopulations in cholangiocarcinomas. Am J Pathol 2015;185:1724-39.
40. Wu HJ, Chu PY. Role of cancer stem cells in cholangiocarcinoma and therapeutic implications. Int J Mol Sci 2019;20:4154.
41. Liang N, Yang T, Huang Q, et al. Mechanism of cancer stemness maintenance in human liver cancer. Cell Death Dis 2022;13:394.
42. Banales JM, Marin JJG, Lamarca A, et al. Cholangiocarcinoma 2020: the next horizon in mechanisms and management. Nat Rev Gastroenterol Hepatol 2020;17:557-88.
43. Vaquero J, Lobe C, Tahraoui S, et al. The IGF2/IR/IGF1R Pathway in tumor cells and myofibroblasts mediates resistance to egfr inhibition in cholangiocarcinoma. Clin Cancer Res 2018;24:4282-96.
44. Lobe C, Vallette M, Arbelaiz A, et al. Zinc finger e-box binding homeobox 1 promotes cholangiocarcinoma progression through tumor dedifferentiation and tumor-stroma paracrine signaling. Hepatology 2021;74:3194-212.
45. Shuang ZY, Wu WC, Xu J, et al. Transforming growth factor-β1-induced epithelial-mesenchymal transition generates ALDH-positive cells with stem cell properties in cholangiocarcinoma. Cancer Lett 2014;354:320-8.
46. Qian Y, Yao W, Yang T, et al. aPKC-ι/P-Sp1/Snail signaling induces epithelial-mesenchymal transition and immunosuppression in cholangiocarcinoma. Hepatology 2017;66:1165-82.
47. Bian J, Fu J, Wang X, et al. Characterization of immunogenicity of malignant cells with stemness in intrahepatic cholangiocarcinoma by single-cell RNA sequencing. Stem Cells Int 2022;2022:3558200.
48. Raggi C, Correnti M, Sica A, et al. Cholangiocarcinoma stem-like subset shapes tumor-initiating niche by educating associated macrophages. J Hepatol 2017;66:102-15.
49. Wang X, Golino JL, Hawk NV, Xie C. Reciprocal interaction of cancer stem cells of cholangiocarcinoma with macrophage. Stem Cell Rev Rep 2023;19:2013-23.
50. Chen Z, Li H, Li Z, et al. SHH/GLI2-TGF-β1 feedback loop between cancer cells and tumor-associated macrophages maintains epithelial-mesenchymal transition and endoplasmic reticulum homeostasis in cholangiocarcinoma. Pharmacol Res 2023;187:106564.
51. Martin-Serrano MA, Kepecs B, Torres-Martin M, et al. Novel microenvironment-based classification of intrahepatic cholangiocarcinoma with therapeutic implications. Gut 2023;72:736-48.
52. Zhang M, Yang H, Wan L, et al. Single-cell transcriptomic architecture and intercellular crosstalk of human intrahepatic cholangiocarcinoma. J Hepatol 2020;73:1118-30.
53. Yang T, Deng Z, Xu L, et al. Macrophages-aPKC(ɩ)-CCL5 Feedback Loop Modulates the Progression and Chemoresistance in Cholangiocarcinoma. J Exp Clin Cancer Res 2022;41:23.
54. Wang X, Eichhorn PJA, Thiery JP. TGF-β, EMT, and resistance to anti-cancer treatment. Semin Cancer Biol 2023;97:1-11.
55. Lin Y, Cai Q, Chen Y, et al. CAFs shape myeloid-derived suppressor cells to promote stemness of intrahepatic cholangiocarcinoma through 5-lipoxygenase. Hepatology 2022;75:28-42.
56. Yang X, Lin Y, Shi Y, et al. FAP promotes immunosuppression by cancer-associated fibroblasts in the tumor microenvironment via STAT3-CCL2 signaling. Cancer Res 2016;76:4124-35.
57. Song G, Shi Y, Meng L, et al. Single-cell transcriptomic analysis suggests two molecularly subtypes of intrahepatic cholangiocarcinoma. Nat Commun 2022;13:1642.
58. Cao L, Bridle KR, Shrestha R, Prithviraj P, Crawford DHG, Jayachandran A. CD73 and PD-L1 as potential therapeutic targets in gallbladder cancer. Int J Mol Sci 2022;23:1565.
59. Greten TF, Schwabe R, Bardeesy N, et al. Immunology and immunotherapy of cholangiocarcinoma. Nat Rev Gastroenterol Hepatol 2023;20:349-65.
60. Tomlinson JL, Valle JW, Ilyas SI. Immunobiology of cholangiocarcinoma. J Hepatol 2023;79:867-75.
61. Paillet J, Kroemer G, Pol JG. Immune contexture of cholangiocarcinoma. Curr Opin Gastroenterol 2020;36:70-6.
62. Job S, Rapoud D, Dos Santos A, et al. Identification of four immune subtypes characterized by distinct composition and functions of tumor microenvironment in intrahepatic cholangiocarcinoma. Hepatology 2020;72:965-81.
63. Kawamoto M, Umebayashi M, Tanaka H, et al. Combined gemcitabine and metronidazole is a promising therapeutic strategy for cancer stem-like cholangiocarcinoma. Anticancer Res 2018;38:2739-48.
64. Tabatabaee A, Nafari B, Farhang A, et al. Targeting vimentin: a multifaceted approach to combatting cancer metastasis and drug resistance. Cancer Metastasis Rev 2023:Online ahead of print.
Cite This Article
Export citation file: BibTeX | RIS
OAE Style
Minini M, Pavy A, Lekbaby B, Fouassier L. Crosstalk between cancer cell plasticity and immune microenvironment in cholangiocarcinoma. Hepatoma Res 2024;10:2. http://dx.doi.org/10.20517/2394-5079.2023.69
AMA Style
Minini M, Pavy A, Lekbaby B, Fouassier L. Crosstalk between cancer cell plasticity and immune microenvironment in cholangiocarcinoma. Hepatoma Research. 2024; 10: 2. http://dx.doi.org/10.20517/2394-5079.2023.69
Chicago/Turabian Style
Minini, Mirko, Allan Pavy, Bouchra Lekbaby, Laura Fouassier. 2024. "Crosstalk between cancer cell plasticity and immune microenvironment in cholangiocarcinoma" Hepatoma Research. 10: 2. http://dx.doi.org/10.20517/2394-5079.2023.69
ACS Style
Minini, M.; Pavy A.; Lekbaby B.; Fouassier L. Crosstalk between cancer cell plasticity and immune microenvironment in cholangiocarcinoma. Hepatoma. Res. 2024, 10, 2. http://dx.doi.org/10.20517/2394-5079.2023.69
About This Article
Special Issue
Copyright
Data & Comments
Data
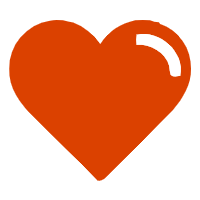

Comments
Comments must be written in English. Spam, offensive content, impersonation, and private information will not be permitted. If any comment is reported and identified as inappropriate content by OAE staff, the comment will be removed without notice. If you have any queries or need any help, please contact us at support@oaepublish.com.