Advanced and emerging radiation therapy approaches for intrahepatic cholangiocarcinoma
Abstract
Radiotherapy (RT) is an integral component of the multidisciplinary care for intrahepatic cholangiocarcinoma (iCCA). Over the past decades, RT techniques have been developed with the aim of enhancing tumor control and minimizing toxicity. The most recent technological advancements include proton beam therapy (PBT) and magnetic resonance-guided radiotherapy (MRgRT). PBT is notable for its unique physical characteristics that allow for greater sparing of surrounding normal organs, especially the liver, from low to moderate doses of radiation. MRgRT provides advantages in other aspects, including superior tumor visualization before treatment, on-board treatment plan adaptation, and tumor tracking during treatment. These features allow for precise dose delivery and safe dose escalation, especially for patients with tumors close to luminal GI structures. In this review article, the rationale, clinical outcomes, clinical applications, challenges, and future directions of PBT and MRgRT are discussed. Additionally, the potential combination of novel therapeutics with RT in iCCA is explored.
Keywords
INTRODUCTION
Intrahepatic cholangiocarcinoma (iCCA) is a relatively rare cancer characterized by an unfavorable long-term survival rate and a high incidence of tumor recurrence[1,2]. To maximize treatment outcomes, a comprehensive, multidisciplinary treatment approach has become essential. Within this approach, radiotherapy (RT) is a component that holds the potential to improve clinical outcomes in select patients.
Conventional RT with photons or x-ray is standard for various types of cancers, including iCCA. However, due to the physical property of photons that result in dose deposition along their trajectory, coupled with the typical anatomic location of iCCA tumors in or near radiosensitive organs such as the liver and luminal GI structures, advanced RT techniques are often necessary for the safe and effective RT treatment delivery.
Over the past decades, radiation techniques have evolved significantly. These advancements aim to optimize the precision of radiation delivery to tumors while minimizing exposure of the surrounding normal tissue. Photon-based radiation techniques have evolved from two-dimensional (2D) techniques to three-dimensional conformal radiotherapy (3D-CRT), to intensity-modulated radiation therapy (IMRT), and most recently, to stereotactic body radiation therapy (SBRT). Each stage of evolution has its advantages. For instance, 3D-CRT, which uses 3D mapping of tumors in place of 2D imaging, enables more dose conformality. However, it lacks the ability to modulate beam intensity - a limitation that IMRT helps overcome. IMRT employs inverse treatment planning, which incorporates defined planning goals to iteratively generate plans that modulate the intensity of radiation beams. SBRT, an even more precise approach, can deliver high radiation doses in five or fewer fractions, enhancing tumor control and reducing toxicity to surrounding tissues.
Concurrently, image-guided radiotherapy (IGRT), an in-room imaging guiding the radiation delivery, has evolved from portable x-ray to orthogonal kilovolt x-ray images, and to cone-beam computed tomography (CBCT). This development enhances the clarity of tumor visualization before each fraction of treatment, enabling more precise radiation delivery with smaller margins.
Despite these advancements, current standard techniques still have limitations in certain situations. Emerging technologies such as proton beam therapy (PBT) and magnetic resonance imaging-guided radiotherapy (MRgRT) have been developed to overcome these limitations. This review examines and summarizes advanced and emerging RT technologies focusing on PBT and MRgRT. Additionally, with advancements in molecular research, targeted therapy and immunotherapy have become the standard of care; we will explore novel RT approaches when combined with these systemic therapies.
ROLE OF RT FOR ICCA
Adjuvant RT for resectable iCCA
Surgical resection is the cornerstone treatment for iCCA. Following surgical resection, adjuvant chemotherapy is considered the standard of care, as it can potentially improve OS[3]. To date, no phase III study has evaluated the efficacy of adjuvant RT or chemoRT (CRT). However, retrospective studies have shown that adjuvant RT may benefit select patients with iCCA[4-9]. The potential benefit of adjuvant CRT is its ability to improve locoregional control or survival, particularly in patients with positive margins or lymph nodes. Kim et al. found that in R0 resection, lymph node-positive iCCA patients, adjuvant CRT was associated with a trend towards longer OS compared with no adjuvant treatment (median OS: 72.1 vs. 27.2 months, P = 0.059), while adjuvant chemotherapy alone did not improve OS[9]. Lin et al. found that in iCCA patients with positive margins, postoperative CRT improved OS compared to adjuvant chemotherapy alone for early (HR 0.65; 95%CI: 0.56-0.92; P = 0.01) and advanced stages (HR 0.51; 95%CI: 0.36-0.75; P < 0.001) patients[7]. Despite studies indicating the potential benefit, the role of adjuvant RT remains controversial as conflicting findings have been reported[10].
The American Society for Radiation Oncology (ASTRO) also conditionally recommends adjuvant CRT, alone or in sequence, after systemic chemotherapy in iCCA patients who underwent curative surgical resection and had high-risk features, including positive lymph nodes and/or R1 resection[11].
RT for localized unresectable iCCA
Although surgery is the main treatment for localized iCCA, only a small percentage of patients are candidates for this treatment option because tumors are often asymptomatic in their early stages. The combination of gemcitabine and cisplatin is considered the standard of care, according to the ABC-02 trial[12]. Recently, the TOPAZ-1 study reported that the addition of durvalumab to this combined chemotherapy may also provide a survival benefit[13,14]. However, most of the patients in both studies had distant metastases[12,13]. For patients with unresectable iCCA without distant organ metastases, locoregional failure remains a problem. Approximately 70% of patients who receive chemotherapy alone die from progressive liver disease and subsequent liver failure rather than distant metastases. Patients who received local therapy showed a lower incidence of liver failure at the time of death, implying that consolidative local therapy may benefit this group of patients[15].
There are several options for locally directed therapy, including catheter-based therapies (chemo- or radioembolization), hepatic arterial infusion of chemotherapy, ablation, and RT, but no randomized controlled trials have compared these modalities. The choice of treatment depends on patient and tumor characteristics and treatment availability and expertise[16].
As chemotherapy has become the standard of care, the question is: "Does additional RT provide benefits in this scenario?" Although there are no randomized studies to answer this question, RT could improve intrahepatic control and reduce the risk of death from liver failure due to intrahepatic tumor progression[15]. Some studies have shown that CRT appears to improve survival outcomes compared with chemotherapy alone[17]. Verma et al. retrospectively reviewed 2,842 patients in the National Cancer Database (NCDB) of newly diagnosed iCCA without distant metastasis: 2,176 (77%) received chemotherapy alone,
Several studies have shown that RT is an effective treatment for localized unresectable iCCA, with 1- and 2-year OS rates ranging from 51%-87% and 31%-62%, respectively[17-25]. LC at 2 years was reported to be between 45%-94%[18,20-23]. Data also suggests a benefit of radiation dose escalation for iCCA tumors. As shown in a retrospective study of inoperable tumors treated with curative intent by Tao et al., a biological equivalent dose (BED10) > 80.5 Gy was associated with improved LC and OS[23]. The 3-year LC for patients who received BED10 > 80.5 Gy was 78%, compared to 45% for those with BED10 ≤ 80.5 Gy (P = 0.04). Additionally, patients with BED10 > 80.5 had superior 3-year OS than those who received BED10 ≤ 80.5 Gy (73% vs. 38%; P = 0.017). De et al. also retrospectively reviewed the data of unresectable iCCA patients in the NCDB and found that patients who received ablative RT with BED10 ≥ 80.5 Gy had significantly better OS than those who received conventional RT with BED10 < 80.5 Gy[19]. The median OS was 23.7 months in the ablative RT group compared to 12.8 months in the conventional RT group (P < 0.001). Ablative RT was an independent prognostic factor for OS (HR 0.6; 95%CI: 0.48-0.76; P < 0.001).
In the context of unresectable iCCA with regional lymph node involvement, systemic treatment is also seen as the standard treatment approach[12,13]. There is limited data available for the use of additional RT or CRT in these cases; however, in theory, these methods might offer improvements in locoregional control. Historical studies conducted prior to the establishment of chemotherapy as a standard treatment indicated that EBRT had a 60% response rate (complete response [CR] and partial response [PR]) in patients with lymph node metastasis and could improve OS in patients with unresectable iCCA (P = 0.003)[26]. These early findings suggest a possible benefit of RT in this patient population, but further research is warranted to evaluate the potential benefits of additional RT.
RT as a neoadjuvant treatment for iCCA
Given that margin-negative resection remains a primary approach in the curative-intent treatment of iCCA, neoadjuvant treatment for patients with localized, unresectable disease can be used to downsize tumors, with the aim of converting these patients to resectable status. This approach shifts the treatment goal from palliative to curative intent. Although neoadjuvant therapy is widely used in other malignancies, it is less commonly applied in patients with iCCA. Currently, there is no high-level evidence supporting the use of neoadjuvant treatment, and comparison data between treatment modalities are absent[27,28]. Our current understanding of radiation in this setting is based primarily on small retrospective studies. One study showed 85.7% of unresectable iCCA patients experiencing tumor shrinkage post CRT, with 71.4% undergoing resection (80% achieving R0 resection), improving OS compared to those who did not undergo surgery (37 months vs. 10 months; P = 0.027)[29]. Another observed 25% PR rate with 12.5% reaching curative resection, leading to better locoregional recurrence-free survival (LRFFS) and OS[30]. These findings suggest that CRT may have a role in downsizing tumors and converting them to a resectable status, which can improve OS[29,30]. Despite these findings, the effectiveness and resectability rates after neoadjuvant treatment can vary significantly, and many questions regarding optimal initial and local treatments, and appropriate radiation doses remain unanswered due to limited data. Further investigations are necessary to understand the role of neoadjuvant EBRT in this setting.
ADVANCED AND EMERGING RADIATION THERAPY TECHNIQUES
Proton beam therapy (PBT) for iCCA
Current standard radiotherapy techniques and limitations
Until recently, the standard RT approach for treating iCCA has involved photon therapy or high-energy
The rationale for PBT
PBT offers a dosimetric advantage over photon RT, providing a promising approach for liver tumor treatment[32,33]. Photon RT deposits doses along the beam path and extends beyond the tumor, leading to undesired exposure from the exit radiation to adjacent normal tissues, especially the healthy liver. On the other hand, proton has a limited range in tissues. The proton energy loss remains minimal until the end of the beam range, with the residual energy lost over a very short distance. This results in a distinct sharp rise in absorbed dose, followed by a rapid dose fall-off, known as the "Bragg peak" distal to the tumor and subsequent sparing of normal tissue exposure to radiation beyond the tumor[34]. Consequently, PBT offers a theoretical clinical advantage over traditional photon-based radiation by enabling safe dose escalation, improved tumor control rates and reduced incidence of acute and late toxicities.
Clinical outcomes and applications of PBT for iCCA
Dose-escalated hypofractionated PBT may lead to improved clinical outcomes, reduced treatment duration, and decreased toxicity compared to photon RT. One-year LC rates range from 67.7% to 97.3%, and the 2-year LC rates vary from 45% to 94.1%. The 1-year OS ranges from 49% to 83%, with 2-year OS rates between 32% and 58%. The incidence of grade 3 or higher toxicity is approximately 10%[20,22,23,35-39]. Relevant studies are summarized in Table 1.
Summary of proton beam therapy (PBT) studies for patients with intrahepatic cholangiocarcinoma (iCCA)
Author | yr | Study type | Country | No.of Pts | RT Details | Pt and tumor characteristic | Median follow-up | LC | OS | G3+ Toxicity |
Hong et al.[20] | 2016 | Prospective phase II study | USA | 83 (iCCA: 37; cHCC/CCA 2) | Treatment volume - GTV = gross tumor - CTV = GTV + (0-10) mm - PTV = CTV + (5-10) mm Dose: peripheral: 67.5 GyE/15 F; central: 58.05 GyE/15 F | Age: median 66.9 yr (range 29.9-87)* Sex: male 35.9%* Solitary lesion: 87.2%* Tumor size: median 6 cm (range 2.2-10.9)* Metastasis: 0%* Cirrhosis: 97.4%* Prior treatment: 61.5% (CT: 61.5%; Resection: 2.6%; Ablation: 2.6%; TACE: 0; Other 23.1%)* | 19.5 mo (range: 0.6-55.9 mo)* | 2-yr LC 94.1% (Recurrence beyond 2 years occurred)* | Median OS 22.5 mo* 1-yr 69.7%* 2-yr 46.5%* | G3: 3 (7.7%): liver failure, stomach ulcer, elevated blirubin* |
Smart et al.[22] | 2020 | Retrospective study | USA | iCCA 66 | Treatment volume - GTV = gross tumor - CTV = GTV + (3-10) mm - PTV = CTV + (5-10) mm Dose: median 58.05 Gy (range 37.5-67.5) in 15 F; median BED of 80.52 Gy (range 46.9-97.9) Technique: - Proton (PS): 32 (48%) - Photon: 34 (52%) | Age: median 76 yr (range 30-92) Sex: male 41% Solitary lesion: 70% Tumor size: 5.6 cm (range 2.5-16 cm) Metastasis: 23% Cirrhosis: 9% Prior treatment: CT: 42%; resection 8% Definitive intent: 77% | 14 mo from RT, 21 mo from diagnosis | 2-yr LC 84% overall; 93% for curative intent | Median OS 25 mo 2-yr OS 58% Curative intent: Median OS 26 mo 2-yr OS 62% | RILD 1 (1.5%); G3+: 7 (11%) |
Tao et al.[23] | 2016 | Retrospective study | USA | iCCA 79 | Treatment volume - GTV = gross tumor - CTV = GTV + (0-10) mm - PTV = CTV + 5 mm) Dose: median 58.05 Gy (range 35-100 Gy) in 3-30 F; median BED 80.05 Gy (range 43.75-180 Gy) Technique: - proton (PS): 25 (32%) - photon: IMRT 41 (52%); 3D-CRT 13 (16%) | Age: median 63 yr (range 31-87) Sex: male 42% Solitary lesion: N/A Tumor diameter: median 7.9 cm (range 2.2-17 cm) Metastasis: 20% Cirrhosis: N/A Prior treatment: chemotherapy 89% | 33 mo (range 11-93 mo) | 1-yr LC 81% 2-yr LC 45% 3-yr LC 27% (89% recur within the high dose RT field) BED10 > 80.5 vs. ≤ 80.5 Gy: 3-yr LC 78% vs. 45% (P = 0.04) | Median OS 30 mo 1-yr OS 87% 2-yr OS 61% 3-yr OS 44% BED10 > 80.5 vs. ≤ 80.5 Gy: 3-yr OS: 73% vs. 38% (P = 0.017) | 0 cases of RILD |
Makita et al.[35] | 2014 | Retrospective study | Japan | 28 (iCCA 6) | Treatment volume - GTV = gross tumor - CTV = GTV + (5-10) mm - PTV = CTV + (5-7) mm Dose: median 68.2 GyE (range 50.6-80 GyE) of 2-3.2 Gy per F; median BED10 75.8 GyE (range, 61.7-105.6 GyE) | Age: median: 71 (range 41-84) Sex: male: 61% Location: iCCA 21%; eCCA 32%; GB 10.7% Solitary lesion: N/A Tumor size: median: 5.2 cm (range 2-17.5 cm) Metastasis: 0% Cirrhosis: N/A Prior treatment: surgery 35.7% Disease status: unresectable: 64.3%; recurrent tumor after surgery: 35.7% | 12 mo (range, 3-29) | 1-yr LC 67.7% BED10 > 70 vs. < 70 GyE: 1-yr LC 83.1% vs. 22.2% (P = 0.002) | 1-yr OS 49% | Acute G3: cholangitis 1 Late G3: cholangitis 2; common bile duct stenosis; duodenal ulcer 1; duodenal hemorrhage 2; duodenal stenosis 1 No G4+ toxicity |
Ohkawa et al.[36] | 2015 | Retrospective study | Japan | iCCA 20 (curative: 12, palliative:8) | Treatment volume - GTV = primary tumor - CTV = GTV + (5-10) mm - PTV = CTV + (5-10) mm Dose: near GI tract 60-77 GyE in 30-35 F; central tumor 72.6-79.2 GyE in 16-22 F; others 55-60 GyE in 10 F; median dose 72.6 GyE in 22 F Technique: double scattering | Age: median: 74 (range 55-82) + Sex: male: 58.3%+ Location: iCCA 100%+ Solitary lesion: 75%+ Tumor size: < 5 cm: 50%; 5.1-9.9 cm: 41.6%; ≥ 10 cm 8.3%+ Metastasis: 0%+ Cirrhosis: N/A+ Prior treatment: N/A+ | 20.8 mo (range, 8.6-62.6) + | 1-yr LC 88%+ 2-yr LC 60%+ 3-yr LC 60%+ | Median OS 27.5 m+ 1-yr OS 82%+ 2-yr OS 61%+ 3-yr OS 38%+ | Acute G3: bone marrow suppression 1 Late G3: biliary tract infection 2 (No G4+) |
Shimizu et al.[37] | 2019 | Retrospective study | Japan | iCCA 37 (25 curatives, 12 palliatives) | Treatment volume - GTV = gross tumor - CTV = GTV + (5-10) mm - PTV = CTV + (5-10) mm Dose: within 2 cm from the GI tract 74 GyE in 37 F; central tumor 72.6 GyE in 22 F; others 66 GyE in 10 F Technique: double scattering | Age: median 72 yr (44-82 yr) + Sex: male 68%+ Solitary lesion: 88%+ Tumor size: median 4.4 cm (range, 1.5-14) + Metastasis: 12%+ CP: A 80%; B 20%+ Prior treatment: N/A+ | 37.5 mo | 1-yr LC 100%+ 2-yr LC 71.5%+ | Median OS 25 m+ 1-yr OS 66.3%+ 2-yr OS 52.4%+ | Acute G3+: 0 Late G3 biliary tract infection = 3* Link to PBT unclear |
Hung et al.[38] | 2020 | Retrospective study | Taiwan | 30 (iCCA 18) | Treatment volume GTV = gross tumor + gross LN CTV = (GTV + 5 mm) + (bile duct + 5-10 mm) Dose: 72.6 GyE in 22 F or 66 GyE in 10; median BED10 96.5 GyE (range 52.7-109.6 GyE) Technique: PS | Age: median 69 yr (range 49-88) Sex: male 50% Location: iCCA 60%; eCCA 30%; GB 10% Solitary lesion: 83.3% Tumor size: 7 cm (range 3.4-17.5 cm) Metastasis: 6.7% Cirrhosis: 6.7% Recurrent disease 16.7% Prior treatment: surgery 6.7%; radiotherapy 10%; induction CT 56.7% | 16 mo (range 3-36 mo) | 1-yr LC 88% | Median OS 19.3 mo 1 yr OS 83% 2 yr OS 32% | G3 dermatitis 2 (6.7%) G3-4 duodenal/gastric ulcer 3 (10%) No grade 5 toxicity RILD 2 (6.7%) |
Kim et al.[39] | 2022 | Retrospective study | Korea | iCCA 47 | Treatment volume - GTV = gross tumor - ITV = GTV + movement of GTV - CTV = ITV - PTV = ITV + (5-7) mm Dose > 2cm from GI organs: 66-80 Gy in 10 F; < 2 cm from GI organs: 45-60 Gy in 10 F; median EQD2 80.0 GyE (54.4-120) Technique: double-scattering | Age: median 67 (45-83) Sex: male 68.1% Solitary lesion: N/A Tumor size: median 5.2 cm (range 1-11 cm) Metastasis: 23.4% Cirrhosis: N/A Prior treatment: systemic treatment 42.6%; TACE 2.1% | 18.3 mo (range 2.4-89.9 mo) | 1-yr LC 91.7% 2-yr LC 86.9% EQD210 ≥ 80 vs. < 80 GyE 2-yr LC 92.7% vs. 66.7% | Median OS 21.9 mo 1 yr OS 63.8% 2 yr OS 42.7% EQD210 ≥ 80 vs. < 80 GyE: 2-yr OS 23.8% vs. 13.2% | G3 toxicity: BC decrease: 2 (4.3%) ilirubin increase 2 (4.3%) No 4+ toxicity |
Several retrospective studies in Asia have supported the feasibility of PBT in this setting[35-39]. Shimizu et al. conducted a retrospective review of 37 patients with previously untreated iCCA treated with PBT[37]. The prescribed dose for curative treatment depended on the tumor location: 74 GyE in 37 fractions
Similar findings were found in another study from Taiwan by Hung et al., using a similar dose regimen:
A Korean study by Kim et al. retrospectively collected data from 47 patients treated with PBT for primary or recurrent iCCA, using a dose of 66-80 GyE in 10 fractions for tumors greater than 2 cm from the GI organs and 45-60 Gy in 10 fractions for tumors less than 2 cm from the GI organs[39]. The median prescribed dose was 63.3 GyE in 10 fractions. The 1-year LC was 91.7% (95%CI: 82.7%-100.7%), and the 2-year LC was 86.9% (95%CI: 74.4%-99.4%). The median OS was 21.9 months (95%CI: 16.2-28.3 months). The rates of OS at 1 and 2 year were 63.8% (95%CI: 50.1%-77.5%) and 42.7% (95%CI: 28.0%-57.4%), respectively. Grade ≥ 3 adverse events were observed in 8.5%.
Studies from the United States also demonstrated the feasibility of PBT. Tao et al. from the MD Anderson Cancer Center reported results in 79 patients with inoperable iCCA treated who were administered definitive RT, with 25 of these patients undergoing PBT[23]. The median tumor size was 7.9 cm (range 2.2-17 cm), and the median RT dose was 58.05 Gy (range 35-100 Gy) in 3-30 fractions, with a median BED10 of 80.5 GyE (range 43.75-180 Gy). The LC rates were 81% at 1 year and 45% at 2 year. The median OS was 30 months, with 1-year and 2-year OS rates of 87% and 61%, respectively. No cases of RILD were observed. A Massachusetts General Hospital Cancer Center study reported the results of 66 patients with recurrent or unresectable iCCA treated with hypofractionated RT. Proton RT was administered to 32 patients, while 34 patients were treated with photon RT. The median dose was 58.05 Gy (range 37.5-67.5 GyE) in 15 fractions, and the median BED10 was 80.52 GyE (range 46.9-97.9 GyE). The median tumor size was 5.6 cm (range 2.5-16 cm). The median follow-up was 14 months. Of the 51 patients who underwent definitive treatment, the 2-year LC rate was 93%, and the OS rate was 62%. A multivariate analysis of OS revealed a tendency for improved survival associated with proton RT, with a HR of 0.5 (P = 0.05). The overall toxicity rate of grade 3 or higher was 11%, and one patient experienced RILD[22].
To date, no phase III randomized controlled trial has compared photon therapy with proton therapy for iCCA. The most robust evidence is provided by a single-arm, phase II multi-institutional study conducted in the United States that included patients with unresectable hepatocellular carcinoma (HCC) or iCCA who underwent 15 fractions of PBT, receiving a maximum total dose of 67.5 GyE[20]. The median dose administered was 58 GyE. Of the 83 patients, 37 had iCCA, and 2 had a mix of HCC/iCCA. With a median follow-up of 19.5 months, the 2-year LC rate was 94.1%, and the 2-year OS rate was 46.5% for iCCA patients. In particular, four additional patients with iCCA experienced recurrence after two years, indicating the possibility of late local failure. Further long-term follow-up is required to determine whether these hypofractionated doses can maintain long-term control. Toxicity of grade 3 or higher was found in 3 patients (7.7%).
Similar to photon-based studies showing potential benefits for radiation dose escalation for iCCA tumors[23], similar results have also been found in studies that have employed PBT for iCCA. The Makita et al. study used PBT to treat 28 patients with recurrent and unresectable CCA, including 6 patients with iCCA[35]. The median dose was 62.8 GyE (range 50.6-80 GyE) with 2-3.2 GyE per fraction and a median BED10 of 75.8 GyE (range 62.7-105.6 GyE). While the 1-year LC rate was 67.7% (95% CI: 56.3%-79.1%), patients who received BED10 > 70 GyE had LC of 83.1%, which was significantly better than the 22.2% LC observed in those with BED10 < 70 GyE (P = 0.002). The study by Kim et al. also showed that EQD210 > 80 GyE was associated with a trend towards higher 2-year freedom from local progression (FFLP) (92.7% vs. 66.7%), progression-free survival (PFS) (11% vs. 4%), and OS (23.8% vs. 13.2%)[39].
Based on these studies, PBT presents benefits in terms of providing favorable outcomes with acceptable toxicity rates. However, a lingering query pertains to identifying the specific patient cohort that would benefit from proton therapy instead of photon therapy. No randomized data are available to address this inquiry. Nevertheless, since ablative dose radiation can potentially result in improved outcomes, the benefit of PBT may be more significant for patients who cannot achieve ablative doses with photon-based RT due to the high dose or volume of RT to organs at risk. Even though cirrhosis may not be present in many patients with iCCA, minimizing the RT dose to the normal liver remains crucial to reducing the risk of RILD. This is particularly important in situations where liver dose cannot meet constraints due to various reasons, such as large tumors, multiple intrahepatic tumors, small liver volume, and/or circumstances that increase the risk of RILD development even with low doses of radiation, such as poor liver function
Figure 1. Case example of a patient with recurrent multifocal iCCA treated with PBT. A 67-yr-old woman initially diagnosed with iCCA was treated with neoadjuvant chemotherapy followed by right hepatic lobectomy, postoperative SBRT to 45 Gy in 5 fractions for close margins (A); and adjuvant chemotherapy. She developed oligometastatic disease and was treated with multiple lines of systemic treatment, with the most recent being ivosidenib. She eventually developed solitary progression in the liver with multifocal intrahepatic recurrence and was treated with salvage PBT with PBS (B); Due to the close proximity of the tumors to nearby GI organs, a simultaneous-integrated boost technique was utilized to safely treat the tumors to 37.5-60 GyE in 15 fractions. She remains disease-progression-free two years after completion of PBT (C).
Challenges and limitations of PBT in iCCA
The characteristic high-dose deposition in the exact range of PBT presents advantages and disadvantages. Because the proton beam range is highly sensitive to variations in tissue electron density within the beam path, tumor underdosing and overdosing of organs at risk are potential concerns. Sources of uncertainty include inaccuracies in Hounsfield unit (HU) values obtained from CT simulation, tumor target uncertainty, organ filling or variation, and breathing motion[40]. However, these uncertainties can be addressed through various methods used during the simulation, treatment planning, and treatment delivery processes.
During CT simulation, uncertainty reduction can be achieved by employing dual-energy CT to enhance HU value accuracy[41], utilizing Monte Carlo dose calculation algorithms to improve dose calculation precision[40], establishing reproducible positions with proper immobilization to minimize setup uncertainty[42], and implementing effective respiratory motion management to mitigate the interplay effects[43]. In the treatment planning phase, uncertainties can be reduced by selecting robust beam angles to circumvent highly variable soft tissue regions and using 3D or 4D robust plan optimization and evaluation[44]. During PBT delivery, plan robustness can be monitored throughout the entire treatment course by using CT-based IGRT or CT-on-rail to detect anatomical changes that could impact the proton range or by utilizing interval quality assurance scans[45].
In addition to these technical limitations of PBT, the availability and cost of PBT are also significant factors to consider. The cost of PBT is higher than that of photon treatment, and PBT machines are not as widely available as photon-based RT. However, the development of compact proton accelerators and single-room gantries may lead to the more widespread availability of proton centers in the future.
Future directions of PBT for iCCA
It is important to note that all clinical results of PBT in iCCA to date have utilized passive scattering techniques[20,22,23,35-39]. In this approach, a narrow proton beam is spread over a larger area to cover the tumor. Although passive scattering provides excellent dose conformity at the distal edge of the tumor, it lacks the ability to conform to the proximal shape of the tumor. PBS proton therapy, particularly intensity-modulated proton therapy (IMPT), represents an advanced technology of PBT. It employs a narrow beam and modulates the intensity of each proton sport to scan across the tumor layer by layer. PBT-IMPT has more potential in its ability to better target conformal regions while sparing critical structures, which may reduce treatment-related toxicities. This technique may be vulnerable to variations in tissue electron density and, consequently, may be less robust than passive scattering proton therapy[46,47]. In addition, it poses more technical challenges for moving targets[48]. However, these issues can be addressed by using proper motion management, 4D robust optimization to improve intrafractional motion robustness, employing rescanning to reduce interplay effects, and performing verification CT more frequently to determine whether adaptive planning is needed[49,50]. Studies using PBS are needed to evaluate the value of this PBT technology for iCCAs.
Proton-FLASH therapy is the latest advancement in RT, garnering attention for its potential to minimize radiation injury to normal tissues while maintaining effective tumor control. In comparison with the conventional dose rate of proton therapy, which is approximately 0.03-0.1Gy/s, FLASH therapy is 400 times more rapid, with a dose rate of > 40 Gy/s[51]. Proton-FLASH has shown promise in superior normal tissue protection and effective tumor control in numerous in vivo studies[52-55]. Consequently, the benefits of Proton-FLASH in terms of normal tissue sparing may be further enhanced. Mascia et al. reported the first-in-human trial of proton-FLASH therapy for the clinical treatment of painful bone metastases in extremities, with results showing clinical feasibility, pain relief similar to conventional dose-rate photon therapy, and minimal toxic effects[56]. In theory, proton-FLASH therapy is an exciting new treatment strategy that can potentially improve cancer treatment using radiation. However, translating this approach into clinical practice remains challenging, and further investigation is necessary.
Magnetic resonance-guided radiation therapy (MRgRT) for iCCA
Current standard radiotherapy techniques and limitations
Delivering an ablative dose to unresectable iCCA using SBRT or hypofractionation is challenging, as it requires accurate tumor targeting to maximize the probability of tumor control while minimizing toxicity. iCCA can be located adjacent to radiosensitive GI organs, such as the stomach, small intestine and duodenum, which have interfractional anatomical changes due to organ filling and peristalsis-induced tumor motion. In addition, the tumor is located in the liver, which moves with respiration. Respiratory motion management should be used, with the need to verify tumor location before treatment delivery. However, high-fidelity visualization is difficult to achieve, as iCCA tumors are often in areas where the tumor cannot be adequately verified with daily imaging using conventional CT-based IGRT due to poor soft tissue resolution and lack of IV contrast. The utilization of fiducial markers is often employed to assist in image guidance. In addition to the drawback of requiring invasive implantation, this approach also has other significant uncertainties, including fiducial marker migration and challenges of imaging and visualizing OAR changes. All these uncertainties may be compensated for by increasing the irradiated target volume, which trades off the potential gain benefit of OAR sparing. Consequently, dose-escalated RT while maintaining the dose to the OARs below the constraint can be difficult to achieve.
The rationale for MRgRT
MRgRT is one of the most promising innovations in RT. These systems have an MRI unit integrated into a linear accelerator (Linac). MRgRT possesses three main advantages: improved soft-tissue visualization, online adaptive radiotherapy (ART), and real-time synchronous tracking and respiratory gating[57-59]. These characteristics offer more precise radiation delivery, which may translate into better clinical outcomes with regard to improved LC and reduced toxicity.
MRgRT enables the implementation of more hypofractionated dose escalation, while minimizing intentional underdosing to the tumor near the OAR by providing precise radiation with a smaller uncertainty margin[60-62]. MRgRT provides better visualization of tumors and OARs than CBCT imaging[63]. Real-time MRI scans can capture intrafractional motion and facilitate tumor tracking. Consequently, an invasive procedure such as fiducial implantation is not required[61,62,64]. Certain MRgRT devices have the capacity to automatically gate delivery, thereby limiting the margin of ITV[65]. As surrounding GI structures have interfraction variation, high-quality images and online ART systems allow the ability to recontour, re-optimize, and adapt treatment to adjust for anatomical changes[66]. Furthermore, MRgRT may also allow for smaller treatment margins to be applied when accounting for motion and setup uncertainties. Together, these features may lead to the potential for improved tumor coverage and reduced dose to normal organs, which may translate to improved tumor local control and decreased normal tissue toxicities.
Clinical outcomes and applications of MRgRT for iCCA
The feasibility and effectiveness of MRgRT in treating iCCA are based on retrospective studies as summarized in Table 2[60,67-69]. Nearly all studies included patients with primary and metastatic liver cancer, except one study that focused only on patients with CCA. Luterstein et al. conducted a retrospective review of 17 patients with CCA (5 with iCCA) treated with MR-guided liver SBRT[68]. The margin of the planning target volume (PTV), which accounts for setup uncertainties, was only 3 mm from the gross tumor volume (GTV), smaller than historically used for treatment planning. The patients were treated with breath-hold respiratory gating and real-time tumor tracking, and adaptive planning was routinely performed. The median RT dose was 40 Gy, delivered in 5 fractions, to tumors with a median tumor size of 25.5 mm (range 14-37 mm). At a median follow-up time of 15.8 months (range 4.5-29.9 months), the LC rates for 1 year and 2 years were 85.6% and 73.3%, respectively. The median OS was 18.5 months, with 1-year and 2-year OS rates of 76% and 46.1%, respectively. Only one patient (6%) who was treated before using adaptive planning experienced grade 3 acute toxicity of duodenal ulcer and perforation. No grade 3 late toxicities were observed. A multi-institutional retrospective study by Rosenberg et al. included 26 patients with primary liver cancer (2 with iCCA) or liver metastasis treated who underwent MR-guided liver SBRT[69]. The median dose delivery was 50 Gy (range 30-60 Gy) in 5 fractions. A 2- to 5-mm expansion was performed to create the PTV. Inspiratory breath hold was used in 16 patients, while 10 patients used a modified shallow internal target volume or exhale-based setup. All of them were monitored with real-time tumor tracking. Daily adaptive treatment was not administered. The median PTV was 98.3 cm3 (range 13-2,034 cm3). At a median follow-up of 21.2 months, the rate of FFLP was 80.4%. Two patients (7.9%) experienced Grade 3 GI toxicity: 1 with hilar stricture and 1 with portal hypertension. There were no reported GI toxicities of grade 4 or higher. Two patients had a decrease in Child-Pugh class. The authors mentioned that both patients had large-volume tumors that led to high mean liver doses.
Summary of magnetic resonance-guided radiotherapy (MRgRT) studies for patients with intrahepatic cholangiocarcinoma (iCCA)
Author | yr | Study type | Country | No.of Pts | RT details | Pt and tumor characteristic | Median follow-up | Local control | OS | Toxicity |
Rogowski et al.[60] | 2021 | Retrospective study | Germany | Primary liver tumor or liver metastasis 11 (iCCA 2) | 0.35 T MR Linac system Treatment volume - GTV = gross tumor - PTV = GTV + (3-5) mm Dose: BED10 - median PTV 84.4 Gy (range 59.5-112.5 Gy) - median GTV 147.9 Gy (range 71.7-200.5 Gy) Motion management - breath-hold respiratory gating ART for all patients Treatment duration: median 53 min (range 46-78) | Age: median 66 (range 47-86) Sex: male 54.5% Type of tumor: liver metastasis 8; intrahepatic recurrence of CCA 2; recurrence of GI stroma tumor at hepatic hilum 1 PTV volume: median 39.1 cm3 (range 8.3-411.3) | 5 mo | no local failure at the time of analysis | N/A | G1: nausea 1 (9%); vomiting 2 (18%); diarrhea 1 (9%) G2+: 0 |
Feldman et al.[67] | 2019 | Retrospective study | USA | Primary liver tumor or liver metastasis 29 (CCA 2) | 0.35 T MR Linac system Treatment volume - GTV = gross tumor - CTV = GTV + region of potential microscopic disease - PTV = CTV + 5 mm Dose: 45-50 Gy in 5 F (except 3 patients treated with 27-42 Gy in 3 F) Motion management -End-exhale: 21 -End-inhale: 6 -Free-breathing: 2 ART: 1 Average treatment times: 34 mins | Sex: male: 65.5% Location: HCC 26; CCA 2; metastatic colon cancer 1 Mean liver dose 5.56 Gy (1.39-10.43) | N/A | N/A | N/A | Nausea/vomiting 1 (3%); abdominal pain with bloody diarrhea 1 (3%) (brief treatment break and resolved without any intervention) |
Luterstein et al.[68] | 2020 | Retrospective study | USA | CCA 17 (iCCA 5) | 0.35 T tri-60Co MRgRT Treatment volume - GTV = gross tumor - CTV = GTV - PTV = CTV + 3 mm Dose: median 40 Gy in 5 F Motion management: breath-hold respiratory gating ART was routinely implemented. | Age: median 57 yr Sex: male 65% Location: hilar 12; intrahepatic 5 Median size: 25.5 mm (range 14-37 mm) | 15.8 mo (range 4.5-29.9) | 1-yr LC 85.6% 2-yr LC 73.3% | median OS 18.5 mo 1-yr OS 76% 2-yr OS 46.1% | Acute toxicity - G1: nausea 8 (47%); fatigue 4 (24%); abdominal pain 2 (12%); shoulder pain 1 (6%) - G2: 0 (0%) - G3: duodenal ulcer with perforation 1 (6%) Late toxicity - G2: gastritis/colitis 1 (6%) |
Rosenberg et al.[69] | 2019 | Multi-institutional retrospective study | USA | Primary liver tumor or liver metastasis 26 (CCA 2) | 0.35 T tri-60Co MRgRT Treatment volume - GTV = gross tumor - CTV = GTV - PTV = CTV + (2-5) mm Dose: 50 Gy (range 30-60 Gy) in 5 F Motion management - Inspiratory breath hold: 16 - Modified shallow internal target volume or exhale-based setup: 10 ART was not common Time in treatment room: 40-60 min (20-30 min of beam-on time) | Age: median 70 yr (range 30-90 yr) Sex: male 65% Primary: HCC 6; lung 3; CCA 2; pancreas 1; sarcoma 1; head and neck 1; others 4 Median PTV: 98.2 cm3 (range 13-2034) CP: A 76.9%; B-C 0%; N/A 23.1% | 21.2 mo | LC 80.4% at 21.2 mo | 1-yr OS 69% 2-yr OS 60% | GI toxicity G3: 2 (8%) G4+ 0 (0%) CP score drop in 2 (8%) |
Chin et al.[70] | 2023 | Retrospective study | USA | Primary liver cancer 99 (CCA or cHCC/CCA 47) | 0.35 T tri-60Co MRgRT 52%; 0.35 T MR Linac system 47% Treatment volume - GTV = gross tumor - CTV = GTV - PTV = CTV + 5 mm Dose: 50 Gy in 5 F Motion management Daily adaptive treatment was considered in patients with tumors located within 2 cm of a luminal GI structure Time in treatment room: N/A Online ART 53% | Age: median 69 (IQR 63-77)* Sex: male 64% Solitary lesion: 81%* Tumor size: median 2.8 cm (IQR 1.7-4.1)* Metastasis: 6%* Systemic treatment: 62%* | 10.1 months* | 1-yr LC 91%* 2-yr LC 91%* | 1-y OS 67% * 2-y OS 31%* | Acute G3+: hepatic 5 (5%;); biliary 6 (6%); GI 6 (6%); AST/ALT 2 (2%); ALP 5 (5%); TB 6 (6%) Late G3+: hepatic 6 (6%); biliary 6 (6%); GI 4 (4%); AST/ALT 5; ALP 8%; TB 11 (11%) |
Rogowski et al. reported 11 patients with primary and secondary liver tumors, 2 of whom had iCCA, treated with online adaptive MRgRT[60]. The PTV expansion was 3-5 mm from the GTV. All patients were treated with breath-hold respiratory gating with real-time tumor tracking. Daily adaptive treatment was done for all patients. The median tumor size was 25 mm (range 14-37 mm), and the median PTV volume was 39.1 cm3 (range 8.3-411.3 cm3). At a median follow-up of 5 months, there were no occurrences of local failure or toxicity at grade 2 or higher. Interestingly, when comparing the nonadaptive and adaptive plans, the results showed that there was less PTV coverage (90.3% vs. 100.2%; P < 0.0001) and more missed PTV
The most recent comprehensive study on the clinical outcomes of MRgRT in primary liver cancer was conducted at Washington University[70]. The study included 99 patients with unresectable primary liver cancer (45 with iCCA and 2 with combined HCC-CCA [cHCC/CCA]), who were treated with MRgRT of 50 Gy in 5 fractions. The PTV margin was defined as a 5 mm expansion from the GTV. Online ART was considered for patients who had tumors situated within 2 cm of luminal GI structures. Among patients with iCCA or cHCC/CCA, 62% had PTV within 1 cm of the duodenum or small bowel and 53% within 1 cm of the stomach. 67% of these patients received online ART. The median follow-up duration was 10.1 months for patients with iCCA or cHCC/CCA, and a 2-year cumulative incidence of local progression was 9.0% (95%CI: 0.1%-18%). The 1- and 2-year OS rates were 67% (95%CI: 53%-84%) and
Regarding clinical application, MRgRT may be more beneficial for iCCA patients whose tumors are located near GI structures such as the stomach, duodenum, and colon. These organs are relatively radiosensitive and are at risk of developing late complications such as bleeding, ulceration, and perforations if exposed to high doses of radiation. Furthermore, they exhibit interfraction variations due to peristalsis and organ filling. Because MRgRT may be able to address these variations and uncertainties at each delivered fraction, escalated doses to the tumor can be more safely delivered. Other scenarios that may potentially benefit from MRgRT include patients with significant motion or those who require fiducial marker implantation but are unable to undergo the procedure. MRgRT has the potential to provide better soft tissue visualization, real-time tumor tracking, and gated treatment in these patients [Figure 2].
Figure 2. Case example of a patient with unresectable iCCA treated with MRgRT. An 81-year-old woman with a pacemaker and cochlear implant, who was diagnosed with a 4 cm iCCA, was initially treated with 3 cycles of gemcitabine and cisplatin followed by MRgRT after stabilization of disease. Due to the proximity of the tumor to the stomach and duodenum, she was treated on a 0.35T MR-Linac and prescribed 50 Gy in 5 consecutive fractions. Since the predicted duodenum and/or stomach dose constraints would have been violated if original plan was used on each fraction due to interfraction anatomic changes, online adaptive replanning was utilized to ensure that all organ at risk constraints were met while optimizing target coverage by the ablative prescription dose. She did not experience acute or late grade 2+ higher toxicity and achieved a complete metabolic response.
Challenges and limitations of MRgRT in iCCA
Although MRgRT represents a significant technological advancement in the field of radiotherapy, further prospective trials are needed to determine its benefits over CT-guided therapy. MRgRT presents challenges in routine clinical implementation, as treatment requires intensive time and resources. The complex workflows and extra steps required for online adaptive MRgRT can extend the total treatment time, which lasts approximately 50-60 mins per adaptive session[60,71]. The process also requires intensive training and coordination of the multidisciplinary team, including radiation therapists, physicists, dosimetrists, and physicians[59,60].
Additionally, substantial investments are required for MRgRT, including upfront costs of the machinery and equipment, staff training, and the longer treatment duration compared to conventional EBRT with other types of IGRT[72]. While the treatment cost of MRgRT is higher, there is no data evaluating its cost-effectiveness for iCCA patients, especially in relation to its potential efficacy improvement and toxicity reduction. Thus, this gap necessitates further research.
The presence of various artifacts, such as those related to metal and motion, also poses additional technical challenges. Motion artifacts can be more challenging for patients who cannot hold their breath. The limited treatment space in MRI-guided machines may lead to difficulty for large patients, those with lateralized tumors and individuals experiencing claustrophobia. Furthermore, the physical structure of the machine restricts the use of multiple beams from different directions, which can potentially reduce the benefit of OAR sparing. Another limitation is that MR-guided machines can track in only one 2D plane, which may be insufficient in some case[73].
In terms of clinical application, while MRgRT offers improved visualization of the tumor and daily treatment plan adaptation, it may still not be able to achieve safe dose escalation in some iCCA patients, such as those with severe cirrhosis or large or multiple tumors. This limitation arises due to MRgRT delivering photon radiation, which has low to moderate dose spillage in the uninvolved liver tissue.
Future directions of MRgRT for iCCA
Fast and accurate auto-segmentation is essential for online adaptive MRgRT. Further development of artificial intelligence could potentially facilitate faster and more accurate auto-segmentation, resulting in enhanced contour quality[74]. When combined with faster automated treatment planning and delivery systems, these may contribute to a shorter MRgRT treatment time, making therapy better tolerated, improving patient compliance, and increasing patient endurance in treatment position[75]. Shortening treatment times could allow more patients to access this advanced technology.
Obtaining a variety of MRI imaging data, which includes both pre-treatment and during-treatment imaging, allows for the investigation of early functional imaging biomarkers and may enable the personalization of RT for individual patients[76]. This approach can potentially lead to administering higher radiation doses to regions of biological resistance while reducing doses in areas with good response, which may result in improved LC while minimizing toxicities.
IMPACT OF MOLECULAR CHARACTERISTICS ON RADIOTHERAPY IN ICCA
Radiation and targeted therapies in iCCA
With the development of gene technologies, the role of gene mutations and abnormal signaling pathways in the pathogenesis of iCCA has been discovered, and several molecular targets have been identified, leading to more treatment opportunities and better treatment outcomes. Fibroblast growth factor receptor 2 (FGFR2) fusion/rearrangement and isocitrate dehydrogenase (IDH) 1 mutation play a significant role in tumorigenesis in iCCA and have become promising targets for treatment. Although FGFR fusion or rearrangement and IDH inhibitors have shown promising efficacy in treating patients with advanced CCA, the impact of these genetic alterations on radiotherapy response remains unclear. In addition to the mutations discussed in this article, there are several other rare mutations in iCCA, including NTRK fusion, BRAF V600E mutation, HER2 overexpression and/or amplification, and RET fusion.
FGFR2 fusion or rearrangement
FGFR is a tyrosine kinase receptor that activates several downstream signaling cascades, leading to cell proliferation. The fusion of FGFR2 results in constitutively active growth factor pathway signaling, promoting tumorigenesis. FGFR2 fusions occur approximately in 20% of iCCAs and are rare in other subsites[77]. Phase II single-arm studies showed that FGFR inhibitors, including pemigatinib, infigratinib and futibatinib, in patients with previously treated, unresectable locally advanced, or metastatic CCA with FGFR2 fusion or other rearrangements, have shown an objective response rate (ORR) of 23.1%-42% and a median PFS of 6.9-9.0 months[78-80].
Data on the effects of FGFR2 fusion on response to radiotherapy, either alone or in combination with an FGFR inhibitor for iCCA, remain limited. Few indirect studies have suggested a potential association between FGFR fusion and radioresistance. Ahmed et al. study demonstrated that FGFR4 mutation inhibited radiation response in colorectal cell lines, and blocking FGFR-4 signaling with FGFR inhibitor increased radiosensitivity[81]. Similarly, Nuryadi et al. also found amplification of FGFR2 in radiotherapy-resistant cervical cancer[82]. Yoshimoto et al. found that patients with FGFR-mutation-positive cervical cancer treated with definitive radiotherapy had significantly worse 5-year PFS compared to FGFR mutation-negative patients (43.9% vs. 68.5%, respectively; P = 0.010)[83].
FGFR inhibitors may potentially increase radiosensitivity. However, the data are limited, and the results are still conflicting. Ader et al. found that an inhibiting FGF-2 pathway by targeting FGFR increased the radiosensitivity of human glioblastoma cells in a preclinical study[84]. In contrast, Verstraete et al. found that FGFR inhibitors did not result in radiosensitization in colorectal cancer cell lines with high expression of FGFR2 either in vitro or in vivo[85].
Currently, there are no available data on FGFR inhibition and RT for iCCA; more research in this area is needed. A phase II study (NCT05565794) investigating the combination of SBRT and pemigatinib in locally advanced iCCA with FGFR fusion or rearrangements is ongoing.
IDH1 mutation
Isocitrate dehydrogenase (IDH) 1 mutation has been observed in approximately 13% of patients with iCCA, with varying frequency and a higher prevalence in non-Asian (less liver fluke-associated) treatment centers. Similar to the FGFR2 fusion, IDH1 mutations are rarely found in eCCA[86]. IDH1 mutation results in the production of the onco-metabolite 2-hydroxyglutarate (2-HG). The accumulation of 2-HG impairs cellular differentiation, leading to tumorigenesis[87]. Ivosidenib, an IDH-1 inhibitor, has been approved by the Food and Drug Administration (FDA) for adults with previously treated, locally advanced, or metastatic CCA with IDH1 mutation. This approval was based on the phase III trial 'ClarIDHy', which involved 185 patients with previously treated advanced IDH1-mutant CCA, comparing ivosidenib with placebo. The study showed a significant increase in the median PFS from 1.4 months using a placebo to 2.7 months with ivosidenib treatment (HR 0.37, 95% CI: 0.25-0.54, P < 0.001)[88].
Most data on radiation response in patients with IDH mutations come from glioma studies, which have shown that IDH1/2 mutant tumors exhibit a better response to radiation[89]. There has been only one preclinical study for iCCA from Wang et al. The study found that IDH mutant iCCA was more sensitive to radiation, both in vitro and preclinical animal models, compared to IDH wild-type[90]. Additionally, the combination of RT and PARP inhibitors (PARPi) in IDH-mutant iCCA led to significantly delayed tumor growth compared to radiotherapy alone. Further clinical investigations are warranted to determine the effect of IDH mutations on radiotherapy response and how radiation can be combined with IDH inhibitors.
Radiation and immunotherapy in iCCA
Although the immune cells have the ability to detect and eradicate aberrant cancer cells, cancer cells have multiple mechanisms to evade immune cells. These mechanisms include adjusting the tumor’s environment to suppress immune reactions through the expression of proteins, such as cytotoxic T-lymphocyte-associated antigen 4 (CTLA-4) and programmed cell death protein 1 (PD-1), and by suppressing MHC expression. Immune checkpoint inhibitors (ICIs), which inhibit interactions between CTLA-4 or PD-1, have demonstrated significant efficacy in treating various cancers. Pembrolizumab, an anti-PD-1 antibody, is FDA-approved for patients with CCA deficient in mismatch repair proteins (dMMR) or with microsatellite instability-high tumors (MSI-H)[91], and for those with a high tumor mutational burden (TMB-H) (≥ 10 mut/Mb)[92]. Notably, dMMR has been reported to occur in approximately 10% of iCCA[93], while the incidence of TMB-H is < 5% of iCCA[94]. Furthermore, the TOPAZ-1 phase III study showed that durvalumab, an anti-PD-L1 antibody in combination with cisplatin and gemcitabine, provided an OS benefit, making this regimen an accepted alternative first-line treatment option for advanced-stage biliary tract cancer in a biomarker-unselected population[13].
The combination of immunotherapy and radiation has shown promising results in many preclinical studies, as it can potentially have a synergistic effect on treatment efficacy. By damaging cancer cells, radiation stimulates the release of tumor-specific antigens that serve as signals, leading to the recognition by immune cells and the activation of cytotoxic T-cells. Radiation can also modulate the tumor microenvironment, potentially enhancing the recruitment and infiltration of immune cells. This concept is the rationale behind the combination of radiation and immunotherapy to eliminate cancer cells[95].
Evidence in the literature on the combination of radiotherapy and ICIs in iCCA has been primarily limited to case reports as summarized in Table 3. Liu et al. reported a case of a 68-year-old male with a low PD-L1 expression level, high MSI, and high TMB stage IV iCCA[96]. Following six cycles of immunotherapy, there was a favorable response in the primary liver and metastatic nodes; however, new lung metastases occurred. Subsequently, the patient underwent radiotherapy for the liver and lung lesions, followed by continued immunotherapy. As a result, a complete response was observed in both the primary tumor and all metastatic sites. A similar result was reported by Zhao et al. in four patients with refractory advanced iCCA or pCCA that were effectively managed with ani-PDL-1 antibody following or concurrent with SBRT[97]. In addition, one patient had a disease that was able to be converted from initially unresectable to resectable after combined therapy.
Summary of a combination of radiotherapy (RT) and immunotherapy studies for patients with intrahepatic cholangiocarcinoma (iCCA)
Author | yr | Study type | Country | No.of Pts | Pt characteristic | Treatment | RT details | Systemic treatment details | Treatment outcomes | Toxicity |
Liu et al.[96] | 2020 | Case report | China | 1 | Patients with stage IV iCCA with low PD-L1 expression, high MSI, and high TMB | After 6 cycles of immunotherapy, mixed response (PD lung, PR liver, and lymph node), so received SBRT to liver and lung lesion, and continued immunotherapy | SBRT with a total dose of 50 Gy to the liver and 48 Gy to the lung | pembrolizumab | CR; The patient patient has survived 26 months after combined treatment and remains tumor-free | No significant toxicity reported |
Zhao et al.[97] | 2021 | Case series | China | 4 | Refractory advanced iCCA or pCCA | Anti-PD-1 antibody following or concurrent with SBRT | SBRT | Nivolumab (n = 2); Pembrolizumab (n = 1); Pembrolizumab + Everoliumus (n = 1) | CR in 1 patient; PR in 2 patients; SD in 1 patient; One unresectable patient became operable | No significant toxicity reported |
Liu et al.[98] | 2019 | Case series | China | 3 | One stage IVA iCCA and 2 postsurgical recurrent iCCA with low TMB, MSS, pMMR, and negative PD-L1 expression | Combined SBRT with PD-1 blocker | SBRT 52-55 Gy in 4-5 Fractions | Nivolumab (n = 1); Pembrolizumab (n = 2) | CR in 1 patient; PR in 2 patients | No significant toxicity reported |
Typically, ICIs provide more responses in patients with dMMR, MSI-H, TMB-H, and/or PD-L1 expression. However, a case report of three advanced-stage or recurrent iCCA patients can benefit from immunotherapy combined with SBRT, even with low TMB, MSS, MMR proficiency, and negative PD-L1 expression[98]. It is possible that radiotherapy may enhance tumor-associated antigen presentation, T-cell recognition, and PD-L1 expression in tumor cells that otherwise may not respond as well to ICI.
Ongoing studies examining the role of combined treatment are summarized in Table 4. The CORRECT trial (NCT03898895), a phase II single-arm study, investigates the combination of RT with camrelizumab, an anti-PD-1 antibody, in patients with unresectable primary or initial postoperative recurrent bile duct cancer without distant metastasis. Another trial, NCT04866836, explores the efficacy and safety of tislelizumab, an anti-PD-1 antibody, combined with RT as a secondary treatment for advanced biliary malignancies in patients who previously received chemotherapy. Another phase I trial (NCT04708067) aims to examine the safety and efficacy of bintrafusp alfa, a bifunctional fusion protein composing the monoclonal antibody avelumab and TGF-beta, in combination with hypofractionated RT for patients with locally advanced or metastatic iCCA who previously received chemotherapy or have refused chemotherapy.
Ongoing studies of a combination of radiotherapy (RT) and immunotherapy for patients with intrahepatic cholangiocarcinoma (iCCA)
Identifier | Phase | Condition | Phase investigating arm | Comparative arm | Radiation dose | Primary outcome | Target accrual |
NCT05565794 | II | Locally advanced iCCA with FGFR fusion or rearrangements | SBRT or other minimally invasive techniques followed by pemigatinib | - | N/A | ORR | 20 |
NCT03898895 | II | Unresectable primary or initial postoperative recurrent bile duct cancer without distant metastasis | RT followed by camrelizumab | - | Total dose over 45 Gy with IMRT or SBRT | PFS | 36 |
NCT04866836 | II | Secondary treatment for advanced biliary malignancies in patients who previously received chemotherapy | RT followed by tislelizumab | - | Total BED over 40 Gy with IMRT or SBRT | ORR | 20 |
NCT04708067 | I | Locally advanced or metastatic iCCA who previously received chemotherapy or have refused chemotherapy. | RT followed by bintrafusp alfa | - | 15 fractions or RT | Adverse events | 15 |
Despite the potential of combined immunotherapy and radiation treatment, numerous challenges remain. The synergistic effect between RT and ICIs is complex and not fully understood, leaving several issues to be addressed. These include determining the optimal volume of irradiation to balance the maximization of RT efficacy and minimization of radiation-induced damage to circulating lymphocytes and the adaptive immune response. Moreover, the ideal sequencing and dose fractionation to stimulate the immune response and overcome resistance is yet to be identified. Ensuring the safety of normal tissues during combined treatment is also a critical concern[99]. Therefore, more research is needed to better understand and optimize this combined treatment approach for iCCA.
CONCLUSION
Radiotherapy can improve LC and possibly OS in the postoperative setting in patients with high-risk features as well as in the locally advanced unresectable setting. Advanced radiotherapy technologies, including PBT and online adaptive MRgRT, can potentially improve treatment outcomes and reduce treatment-related toxicities in iCCA. PBT has a favorable depth-dose characteristic with the Bragg peak, which can reduce low-dose spillage to surrounding organs, thereby increasing the possibility of dose escalation while minimizing toxicity. The benefits of PBT may be more pronounced in patients with large tumors, multifocal disease, or poor baseline liver function.
The advantages of adaptive MRgRT include better tumor localization and tracking during treatment, as well as online adaptation. These features enable treatment with smaller PTV margins, facilitating safe dose escalation while maintaining the dose to the OAR within constraints. This benefit may be more pronounced in patients with tumors in close proximity to other GI structures. Although these advanced technologies show potential benefits, their cost and accessibility are relevant challenges that will need to be addressed. Additional research is needed to validate their efficacy and toxicity in comparison with other treatment modalities.
In the era of advanced molecular technology, targeted therapies and immunotherapy are rapidly developing and altering the treatment approach for many patients diagnosed with advanced-stage iCCA. Further research is warranted to maximize the efficacy of the combination of RT and immunotherapy and to understand its implications.
DECLARATIONS
Authors’ contributions
Made substantial contributions to the conception and design of the study and performed data analysis and interpretation: Thonglert K, Chuong MD, Herrera R, Apisarnthanarax S
Availability of data and materials
Not applicable.
Financial support and sponsorship
None.
Conflicts of interest
All authors declared that there are no conflicts of interest.
Ethical approval and consent to participate
Not applicable.
Consent for publication
Not applicable.
Copyright
© The Author(s) 2023.
REFERENCES
1. Rumgay H, Ferlay J, de Martel C, et al. Global, regional and national burden of primary liver cancer by subtype. Eur J Cancer 2022;161:108-18.
2. Banales JM, Marin JJG, Lamarca A, et al. Cholangiocarcinoma 2020: the next horizon in mechanisms and management. Nat Rev Gastroenterol Hepatol 2020;17:557-88.
3. Primrose JN, Fox RP, Palmer DH, et al. BILCAP study group. Capecitabine compared with observation in resected biliary tract cancer (BILCAP): a randomised, controlled, multicentre, phase 3 study. Lancet Oncol 2019;20:663-73.
4. Zheng X, Chen B, Wu JX, et al. Benefit of adjuvant radiotherapy following narrow-margin hepatectomy in patients with intrahepatic cholangiocarcinoma that adhere to major vessels. Cancer Manag Res 2018;10:3973-81.
5. Jiang W, Zeng ZC, Tang ZY, et al. Benefit of radiotherapy for 90 patients with resected intrahepatic cholangiocarcinoma and concurrent lymph node metastases. J Cancer Res Clin Oncol 2010;136:1323-31.
6. Sur MD, In H, Sharpe SM, et al. Defining the benefit of adjuvant therapy following resection for intrahepatic cholangiocarcinoma. Ann Surg Oncol 2015;22:2209-17.
7. Lin YK, Hsieh MC, Wang WW, et al. Outcomes of adjuvant treatments for resectable intrahepatic cholangiocarcinoma: chemotherapy alone, sequential chemoradiotherapy, or concurrent chemoradiotherapy. Radiother Oncol 2018;128:575-83.
8. Shinohara ET, Mitra N, Guo M, Metz JM. Radiation therapy is associated with improved survival in the adjuvant and definitive treatment of intrahepatic cholangiocarcinoma. Int J Radiat Oncol Biol Phys 2008;72:1495-501.
9. Kim YS, Oh SY, Go SI, et al. The role of adjuvant therapy after R0 resection for patients with intrahepatic and perihilar cholangiocarcinomas. Cancer Chemother Pharmacol 2017;79:99-106.
10. Hammad AY, Berger NG, Eastwood D, et al. Is radiotherapy warranted following intrahepatic cholangiocarcinoma resection? the impact of surgical margins and lymph node status on survival. Ann Surg Oncol 2016;23:912-20.
11. Apisarnthanarax S, Barry A, Cao M, et al. External beam radiation therapy for primary liver cancers: an astro clinical practice guideline. Pract Radiat Oncol 2022;12:28-51.
12. Valle J, Wasan H, Palmer DH, et al. ABC-02 Trial Investigators. Cisplatin plus gemcitabine versus gemcitabine for biliary tract cancer. N Engl J Med 2010;362:1273-81.
13. Oh D, Ruth He A, Qin S, et al. Durvalumab plus gemcitabine and cisplatin in advanced biliary tract cancer. NEJM Evid 2022;1:EVIDoa2200015.
14. Oh DY, He AR, Qin S, et al. 56P updated overall survival (OS) from the phase III TOPAZ-1 study of durvalumab (D) or placebo (PBO) plus gemcitabine and cisplatin (+ GC) in patients (pts) with advanced biliary tract cancer (BTC). Ann Oncol 2022;33:S565-6.
15. Yamashita S, Koay EJ, Passot G, et al. Local therapy reduces the risk of liver failure and improves survival in patients with intrahepatic cholangiocarcinoma: a comprehensive analysis of 362 consecutive patients. Cancer 2017;123:1354-62.
17. Verma V, Kusi Appiah A, Lautenschlaeger T, Adeberg S, Simone CB 2nd, Lin C. Chemoradiotherapy versus chemotherapy alone for unresected intrahepatic cholangiocarcinoma: practice patterns and outcomes from the national cancer data base. J Gastrointest Oncol 2018;9:527-35.
18. Brunner TB, Blanck O, Lewitzki V, et al. Stereotactic body radiotherapy dose and its impact on local control and overall survival of patients for locally advanced intrahepatic and extrahepatic cholangiocarcinoma. Radiother Oncol 2019;132:42-7.
19. De B, Tran Cao HS, Vauthey JN, et al. Ablative liver radiotherapy for unresected intrahepatic cholangiocarcinoma: patterns of care and survival in the United States. Cancer 2022;128:2529-39.
20. Hong TS, Wo JY, Yeap BY, et al. Multi-institutional phase II study of high-dose hypofractionated proton beam therapy in patients with localized, unresectable hepatocellular carcinoma and intrahepatic cholangiocarcinoma. J Clin Oncol 2016;34:460-8.
21. Mahadevan A, Dagoglu N, Mancias J, et al. Stereotactic body radiotherapy (SBRT) for intrahepatic and hilar cholangiocarcinoma. J Cancer 2015;6:1099-104.
22. Smart AC, Goyal L, Horick N, et al. Hypofractionated radiation therapy for unresectable/locally recurrent intrahepatic cholangiocarcinoma. Ann Surg Oncol 2020;27:1122-9.
23. Tao R, Krishnan S, Bhosale PR, et al. Ablative radiotherapy doses lead to a substantial prolongation of survival in patients with inoperable intrahepatic cholangiocarcinoma: a retrospective dose response analysis. J Clin Oncol 2016;34:219-26.
24. Tse RV, Hawkins M, Lockwood G, et al. Phase I study of individualized stereotactic body radiotherapy for hepatocellular carcinoma and intrahepatic cholangiocarcinoma. J Clin Oncol 2008;26:657-64.
25. Weiner AA, Olsen J, Ma D, et al. Stereotactic body radiotherapy for primary hepatic malignancies-report of a phase I/II institutional study. Radiother Oncol 2016;121:79-85.
26. Pascher A, Jonas S, Neuhaus P. Intrahepatic cholangiocarcinoma: indication for transplantation. J Hepatobiliary Pancreat Surg 2003;10:282-7.
27. Chen X, Du J, Huang J, Zeng Y, Yuan K. Neoadjuvant and adjuvant therapy in intrahepatic cholangiocarcinoma. J Clin Transl Hepatol 2022;10:553-63.
28. Akateh C, Ejaz AM, Pawlik TM, Cloyd JM. Neoadjuvant treatment strategies for intrahepatic cholangiocarcinoma. World J Hepatol 2020;12:693-708.
29. Sumiyoshi T, Shima Y, Okabayashi T, et al. Chemoradiotherapy for initially unresectable locally advanced cholangiocarcinoma. World J Surg 2018;42:2910-8.
30. Cho Y, Kim TH, Seong J. Improved oncologic outcome with chemoradiotherapy followed by surgery in unresectable intrahepatic cholangiocarcinoma. Strahlenther Onkol 2017;193:620-9.
31. Chuong MD, Kaiser A, Khan F, et al. Consensus report from the miami liver proton therapy conference. Front Oncol 2019;9:457.
32. Wang X, Krishnan S, Zhang X, et al. Proton radiotherapy for liver tumors: dosimetric advantages over photon plans. Med Dosim 2008;33:259-67.
33. Petersen JB, Lassen Y, Hansen AT, Muren LP, Grau C, Høyer M. Normal liver tissue sparing by intensity-modulated proton stereotactic body radiotherapy for solitary liver tumours. Acta Oncol 2011;50:823-8.
34. Engelsman M, Schwarz M, Dong L. Physics controversies in proton therapy. Semin Radiat Oncol 2013;23:88-96.
35. Makita C, Nakamura T, Takada A, et al. Clinical outcomes and toxicity of proton beam therapy for advanced cholangiocarcinoma. Radiat Oncol 2014;9:26.
36. Ohkawa A, Mizumoto M, Ishikawa H, et al. Proton beam therapy for unresectable intrahepatic cholangiocarcinoma. J Gastroenterol Hepatol 2015;30:957-63.
37. Shimizu S, Okumura T, Oshiro Y, et al. Clinical outcomes of previously untreated patients with unresectable intrahepatic cholangiocarcinoma following proton beam therapy. Radiat Oncol 2019;14:241.
38. Hung SP, Huang BS, Hsieh CE, et al. Clinical outcomes of patients with unresectable cholangiocarcinoma treated with proton beam therapy. Am J Clin Oncol 2020;43:180-6.
39. Kim TH, Woo SM, Lee WJ, et al. Clinical efficacy of hypofractionated proton beam therapy for intrahepatic cholangiocarcinoma. Cancers 2022;14:5561.
40. Tryggestad EJ, Liu W, Pepin MD, Hallemeier CL, Sio TT. Managing treatment-related uncertainties in proton beam radiotherapy for gastrointestinal cancers. J Gastrointest Oncol 2020;11:212-24.
41. Bär E, Lalonde A, Royle G, Lu HM, Bouchard H. The potential of dual-energy CT to reduce proton beam range uncertainties. Med Phys 2017;44:2332-44.
42. Wroe AJ, Bush DA, Slater JD. Immobilization considerations for proton radiation therapy. Technol Cancer Res Treat 2014;13:217-26.
44. Liu W, Zhang X, Li Y, Mohan R. Robust optimization of intensity modulated proton therapy. Med Phys 2012;39:1079-91.
45. Hu YH, Harper RH, Deiter NC, et al. Analysis of the rate of re-planning in spot-scanning proton therapy. Int J Part Ther 2022;9:49-58.
46. Lomax AJ. Intensity modulated proton therapy and its sensitivity to treatment uncertainties 2: the potential effects of inter-fraction and inter-field motions. Phys Med Biol 2008;53:1043-56.
47. Lomax AJ. Intensity modulated proton therapy and its sensitivity to treatment uncertainties 1: the potential effects of calculational uncertainties. Phys Med Biol 2008;53:1027-42.
48. Bert C, Grözinger SO, Rietzel E. Quantification of interplay effects of scanned particle beams and moving targets. Phys Med Biol 2008;53:2253-65.
49. Zhang Y, Boye D, Tanner C, Lomax AJ, Knopf A. Respiratory liver motion estimation and its effect on scanned proton beam therapy. Phys Med Biol 2012;57:1779-95.
50. De Ruysscher D, Sterpin E, Haustermans K, Depuydt T. Tumour movement in proton therapy: solutions and remaining questions: a review. Cancers 2015;7:1143.
51. Lin B, Gao F, Yang Y, et al. FLASH radiotherapy: history and future. Front Oncol 2021;11:644400.
52. Montay-Gruel P, Acharya MM, Petersson K, et al. Long-term neurocognitive benefits of FLASH radiotherapy driven by reduced reactive oxygen species. Proc Natl Acad Sci USA 2019;116:10943-51.
53. Vozenin MC, De Fornel P, Petersson K, et al. The advantage of FLASH radiotherapy confirmed in mini-pig and cat-cancer patients. Clin Cancer Res 2019;25:35-42.
54. Favaudon V, Caplier L, Monceau V, et al. Ultrahigh dose-rate FLASH irradiation increases the differential response between normal and tumor tissue in mice. Sci Transl Med 2014;6:245ra93.
55. Ruan JL, Lee C, Wouters S, et al. Irradiation at ultra-high (FLASH) dose rates reduces acute normal tissue toxicity in the mouse gastrointestinal system. Int J Radiat Oncol Biol Phys 2021;111:1250-61.
56. Mascia AE, Daugherty EC, Zhang Y, et al. Proton FLASH radiotherapy for the treatment of symptomatic bone metastases: the FAST-01 nonrandomized trial. JAMA Oncol 2023;9:62-9.
57. Raaymakers BW, Lagendijk JJ, Overweg J, et al. Integrating a 1.5 T MRI scanner with a 6 MV accelerator: proof of concept. Phys Med Biol 2009;54:N229-37.
58. Mutic S, Dempsey JF. The viewray system: magnetic resonance-guided and controlled radiotherapy. Semin Radiat Oncol 2014;24:196-9.
59. Raaymakers BW, Jürgenliemk-Schulz IM, Bol GH, et al. First patients treated with a 1.5 T MRI-Linac: clinical proof of concept of a high-precision, high-field MRI guided radiotherapy treatment. Phys Med Biol 2017;62:L41-50.
60. Rogowski P, von Bestenbostel R, Walter F, et al. Feasibility and early clinical experience of online adaptive mr-guided radiotherapy of liver tumors. Cancers 2021;13:1523.
61. Klüter S, Katayama S, Spindeldreier CK, et al. First prospective clinical evaluation of feasibility and patient acceptance of magnetic resonance-guided radiotherapy in Germany. Strahlenther Onkol 2020;196:691-8.
62. Corradini S, Alongi F, Andratschke N, et al. MR-guidance in clinical reality: current treatment challenges and future perspectives. Radiat Oncol 2019;14:92.
63. Noel CE, Parikh PJ, Spencer CR, et al. Comparison of onboard low-field magnetic resonance imaging versus onboard computed tomography for anatomy visualization in radiotherapy. Acta Oncol 2015;54:1474-82.
64. Kurz C, Buizza G, Landry G, et al. Medical physics challenges in clinical MR-guided radiotherapy. Radiat Oncol 2020;15:93.
65. Mittauer K, Paliwal B, Hill P, et al. A new era of image guidance with magnetic resonance-guided radiation therapy for abdominal and thoracic malignancies. Cureus 2018;10:e2422.
66. Henke L, Kashani R, Robinson C, et al. Phase I trial of stereotactic MR-guided online adaptive radiation therapy (SMART) for the treatment of oligometastatic or unresectable primary malignancies of the abdomen. Radiother Oncol 2018;126:519-26.
67. Feldman AM, Modh A, Glide-Hurst C, Chetty IJ, Movsas B. Real-time magnetic resonance-guided liver stereotactic body radiation therapy: an institutional report using a magnetic resonance-linac system. Cureus 2019;11:e5774.
68. Luterstein E, Cao M, Lamb JM, et al. Clinical outcomes using magnetic resonance-guided stereotactic body radiation therapy in patients with locally advanced cholangiocarcinoma. Adv Radiat Oncol 2019;5:189-95.
69. Rosenberg SA, Henke LE, Shaverdian N, et al. A multi-institutional experience of mr-guided liver stereotactic body radiation therapy. Adv Radiat Oncol 2019;4:142-9.
70. Chin RI, Schiff JP, Bommireddy A, et al. Clinical outcomes of patients with unresectable primary liver cancer treated with MR-guided stereotactic body radiation therapy: a Six-Year experience. Clin Transl Radiat Oncol 2023;41:100627.
71. Lamb J, Cao M, Kishan A, et al. Online adaptive radiation therapy: implementation of a new process of care. Cureus 2017;9:e1618.
72. Hall WA, Paulson E, Li XA, et al. Magnetic resonance linear accelerator technology and adaptive radiation therapy: an overview for clinicians. CA Cancer J Clin 2022;72:34-56.
73. Witt JS, Rosenberg SA, Bassetti MF. MRI-guided adaptive radiotherapy for liver tumours: visualising the future. Lancet Oncol 2020;21:e74-82.
74. Ding J, Zhang Y, Amjad A, et al. Deep learning based automatic contour refinement for inaccurate auto-segmentation in MR-guided adaptive radiotherapy. Phys Med Biol 2023;68:055004.
75. Güngör G, Serbez İ, Temur B, et al. Time analysis of online adaptive magnetic resonance-guided radiation therapy workflow according to anatomical sites. Pract Radiat Oncol 2021;11:e11-21.
76. van Houdt PJ, Yang Y, van der Heide UA, et al. Quantitative magnetic resonance imaging for biological image-guided adaptive radiotherapy. Front Oncol 2020;10:615643.
77. Goyal L, Shi L, Liu LY, et al. TAS-120 overcomes resistance to ATP-competitive FGFR inhibitors in patients with FGFR2 fusion-positive intrahepatic cholangiocarcinoma. Cancer Discov 2019;9:1064-79.
78. Javle M, Roychowdhury S, Kelley RK, et al. Infigratinib (BGJ398) in previously treated patients with advanced or metastatic cholangiocarcinoma with FGFR2 fusions or rearrangements: mature results from a multicentre, open-label, single-arm, phase 2 study. Lancet Gastroenterol Hepatol 2021;6:803-15.
79. Abou-Alfa GK, Sahai V, Hollebecque A, et al. Pemigatinib for previously treated, locally advanced or metastatic cholangiocarcinoma: a multicentre, open-label, phase 2 study. Lancet Oncol 2020;21:671-84.
80. Goyal L, Meric-Bernstam F, Hollebecque A, et al. FOENIX-CCA2 Study Investigators. Futibatinib for FGFR2-rearranged intrahepatic cholangiocarcinoma. N Engl J Med 2023;388:228-39.
81. Ahmed MA, Selzer E, Dörr W, et al. Fibroblast growth factor receptor 4 induced resistance to radiation therapy in colorectal cancer. Oncotarget 2016;7:69976-90.
82. Nuryadi E, Sasaki Y, Hagiwara Y, et al. Mutational analysis of uterine cervical cancer that survived multiple rounds of radiotherapy. Oncotarget 2018;9:32642-52.
83. Yoshimoto Y, Sasaki Y, Murata K, et al. Mutation profiling of uterine cervical cancer patients treated with definitive radiotherapy. Gynecol Oncol 2020;159:546-53.
84. Ader I, Delmas C, Skuli N, et al. Preclinical evidence that SSR128129E--a novel small-molecule multi-fibroblast growth factor receptor blocker--radiosensitises human glioblastoma. Eur J Cancer 2014;50:2351-9.
85. Verstraete M, Debucquoy A, Gonnissen A, et al. In vitro and in vivo evaluation of the radiosensitizing effect of a selective FGFR inhibitor (JNJ-42756493) for rectal cancer. BMC Cancer 2015;15:946.
86. Boscoe AN, Rolland C, Kelley RK. Frequency and prognostic significance of isocitrate dehydrogenase 1 mutations in cholangiocarcinoma: a systematic literature review. J Gastrointest Oncol 2019;10:751-65.
87. Dang L, White DW, Gross S, et al. Cancer-associated IDH1 mutations produce 2-hydroxyglutarate. Nature 2009;462:739-44.
88. Abou-Alfa GK, Macarulla T, Javle MM, et al. Ivosidenib in IDH1-mutant, chemotherapy-refractory cholangiocarcinoma (ClarIDHy): a multicentre, randomised, double-blind, placebo-controlled, phase 3 study. Lancet Oncol 2020;21:796-807.
89. Tran AN, Lai A, Li S, et al. Increased sensitivity to radiochemotherapy in IDH1 mutant glioblastoma as demonstrated by serial quantitative MR volumetry. Neuro Oncol 2014;16:414-20.
90. Wang Y, Wild AT, Turcan S, et al. Targeting therapeutic vulnerabilities with PARP inhibition and radiation in IDH-mutant gliomas and cholangiocarcinomas. Sci Adv 2020;6:eaaz3221.
91. FDA grants accelerated approval to pembrolizumab for first tissue/site agnostic indication. Available from: https://www.fda.gov/drugs/resources-information-approved-drugs/fda-grants-accelerated-approval-pembrolizumab-first-tissuesite-agnostic-indication [Last accessed on 15 Aug 2023].
92. FDA approves pembrolizumab for adults and children with TMB-H solid tumors. Available from: https://www.fda.gov/drugs/drug-approvals-and-databases/fda-approves-pembrolizumab-adults-and-children-tmb-h-solid-tumors [Last accessed on 15 Aug 2023].
93. Silva VW, Askan G, Daniel TD, et al. Biliary carcinomas: pathology and the role of DNA mismatch repair deficiency. Chin Clin Oncol 2016;5:62.
94. Israel MA, Danziger N, McGregor KA, et al. Comparative genomic analysis of intrahepatic cholangiocarcinoma: biopsy type, ancestry, and testing patterns. Oncologist 2021;26:787-96.
95. Wang Y, Deng W, Li N, et al. Combining immunotherapy and radiotherapy for cancer treatment: current challenges and future directions. Front Pharmacol 2018;9:185.
96. Liu ZL, Liu X, Peng H, et al. Anti-PD-1 immunotherapy and radiotherapy for stage IV intrahepatic cholangiocarcinoma: a case report. Front Med 2020;7:368.
97. Zhao Q, Chen Y, Du S, et al. Integration of radiotherapy with anti-PD-1 antibody for the treatment of intrahepatic or hilar cholangiocarcinoma: reflection from four cases. Cancer Biol Ther 2021;22:175-83.
98. Liu X, Yao J, Song L, Zhang S, Huang T, Li Y. Local and abscopal responses in advanced intrahepatic cholangiocarcinoma with low TMB, MSS, pMMR and negative PD-L1 expression following combined therapy of SBRT with PD-1 blockade. J Immunother Cancer 2019;7:204.
Cite This Article
Export citation file: BibTeX | RIS
OAE Style
Thonglert K, Chuong MD, Herrera R, Apisarnthanarax S. Advanced and emerging radiation therapy approaches for intrahepatic cholangiocarcinoma. Hepatoma Res 2023;9:40. http://dx.doi.org/10.20517/2394-5079.2023.47
AMA Style
Thonglert K, Chuong MD, Herrera R, Apisarnthanarax S. Advanced and emerging radiation therapy approaches for intrahepatic cholangiocarcinoma. Hepatoma Research. 2023; 9: 40. http://dx.doi.org/10.20517/2394-5079.2023.47
Chicago/Turabian Style
Thonglert, Kanokphorn, Michael D. Chuong, Robert Herrera, Smith Apisarnthanarax. 2023. "Advanced and emerging radiation therapy approaches for intrahepatic cholangiocarcinoma" Hepatoma Research. 9: 40. http://dx.doi.org/10.20517/2394-5079.2023.47
ACS Style
Thonglert, K.; Chuong MD.; Herrera R.; Apisarnthanarax S. Advanced and emerging radiation therapy approaches for intrahepatic cholangiocarcinoma. Hepatoma. Res. 2023, 9, 40. http://dx.doi.org/10.20517/2394-5079.2023.47
About This Article
Special Issue
Copyright
Data & Comments
Data
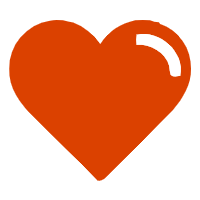

Comments
Comments must be written in English. Spam, offensive content, impersonation, and private information will not be permitted. If any comment is reported and identified as inappropriate content by OAE staff, the comment will be removed without notice. If you have any queries or need any help, please contact us at support@oaepublish.com.